Microfluidics in Drug Delivery: A New Era of Precision Medicine
Microfluidics in drug delivery is emerging as a transformative approach, enabling the miniaturization and automation of complex laboratory processes on chip-scale platforms. These systems offer precise dosing and enable the creation of drug carriers designed for site-specific delivery and controlled release.
In this review, we will discuss how microfluidics in drug delivery is advancing the fabrication of lipid-based and polymeric carriers, as well as its application in microneedle systems for efficient, minimally invasive drug delivery. We will also explore the role of microfluidic platforms in drug crystallization, which aids in structural analysis and optimization of drug formulations. These advancements are key for improving drug delivery systems.
Table of contents:
How Does Microfluidics Compare Classical Methods in Drug Delivery?
Traditional drug delivery methods, including oral administration, injections, and inhalation, have long depended on conventional techniques to fabricate drug carriers such as liposomes and polymeric nanoparticles.1,2 Although these systems aim to enhance drug solubility, stability, and targeting, their fabrication processes are often complex, difficult to scale, and limited in precision and reproducibility. Combined with challenges like poor biodistribution and biological barriers, these limitations reduce the effectiveness of many therapeutic agents.3
Microfluidic technology in healthcare offers a promising shift toward more efficient and controlled delivery systems. Known for its ability to manipulate fluids at the microscale, microfluidics enables the precise, scalable, and reproducible production of advanced drug carriers (Figure 1).4–6
This review explores recent developments in precision drug delivery using microfluidics, structured into three main areas:
(a) the fabrication of drug carriers, including lipid-based and polymeric nanoparticles, through microfluidic platforms;
(b) the integration of microfluidics into microneedle technologies for minimally invasive applications;
(c) the broader applications of microfluidics in nanomedicine and drug research, such as crystallization techniques and the development of in vitro platforms for studying delivery mechanisms.
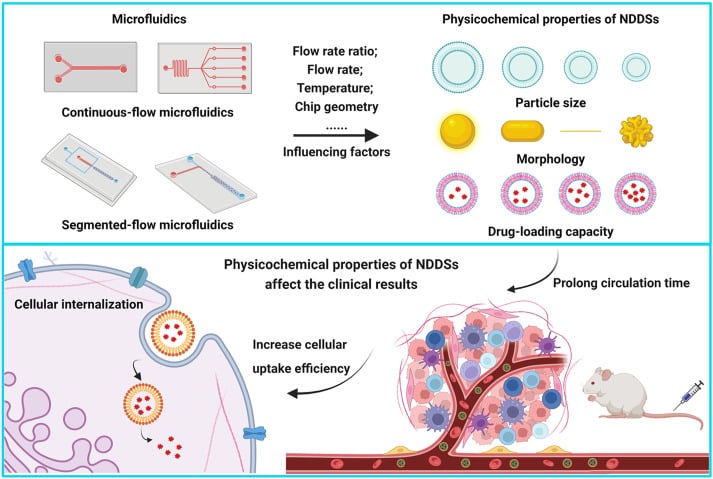
From Zhang, H. et al.; Acta Pharmaceutica Sinica B 2023, 13 (8), 3277–3299).
How Do Microfluidic Techniques Improve Drug Delivery Particle Synthesis?
1- Microfluidic Techniques for Lipid Nanoparticle Synthesis:
Lipid-based nanoparticles (LNPs) are a promising class of drug delivery systems due to their biocompatibility, capacity for encapsulating diverse therapeutic agents, and ability to facilitate controlled drug release. LNPs are particularly useful for delivering hydrophobic drugs, nucleic acids, and other sensitive molecules. Here are some microfluidic techniques used in LNP synthesis for drug delivery:7
- Microfluidic Hydrodynamic Focusing (MHF):
This technique uses a central stream of lipids dissolved in solvent, bordered by aqueous buffer streams. As the lipid solution is focused into a thin flow, rapid diffusion occurs, triggering self-assembly of nanoparticles (Figure 2-A). The flow rate ratio (FRR) between streams determines the extent of focusing, allowing precise control over particle characteristics. MHF also allows simultaneous encapsulation of hydrophilic drugs by adjusting the stream configurations.8,9
- Chaotic Advection Mixers:
These systems incorporate specially designed microchannels, such as herringbone patterns or serpentine paths, that disrupt laminar flow and induce rapid mixing through stretching and folding of fluid layers (Figure 2-B). This ensures that solvents and lipids mix quickly, initiating nanoparticle formation with improved uniformity. Chaotic advection combines the benefits of fast mixing with continuous-flow operation. 10–12. Explore the full potential of liposome production using this method for drug delivery.
- Vortex Focusing:
A hybrid of MHF and chaotic mixing, this approach uses a conical chamber where the lipid solution enters axially, and the buffer enters tangentially (Figure 2-C). The resulting spiral flow simultaneously focuses on the lipid stream and enhances mixing through rotational motion, enabling efficient nanoparticle formation in a single step.13,14
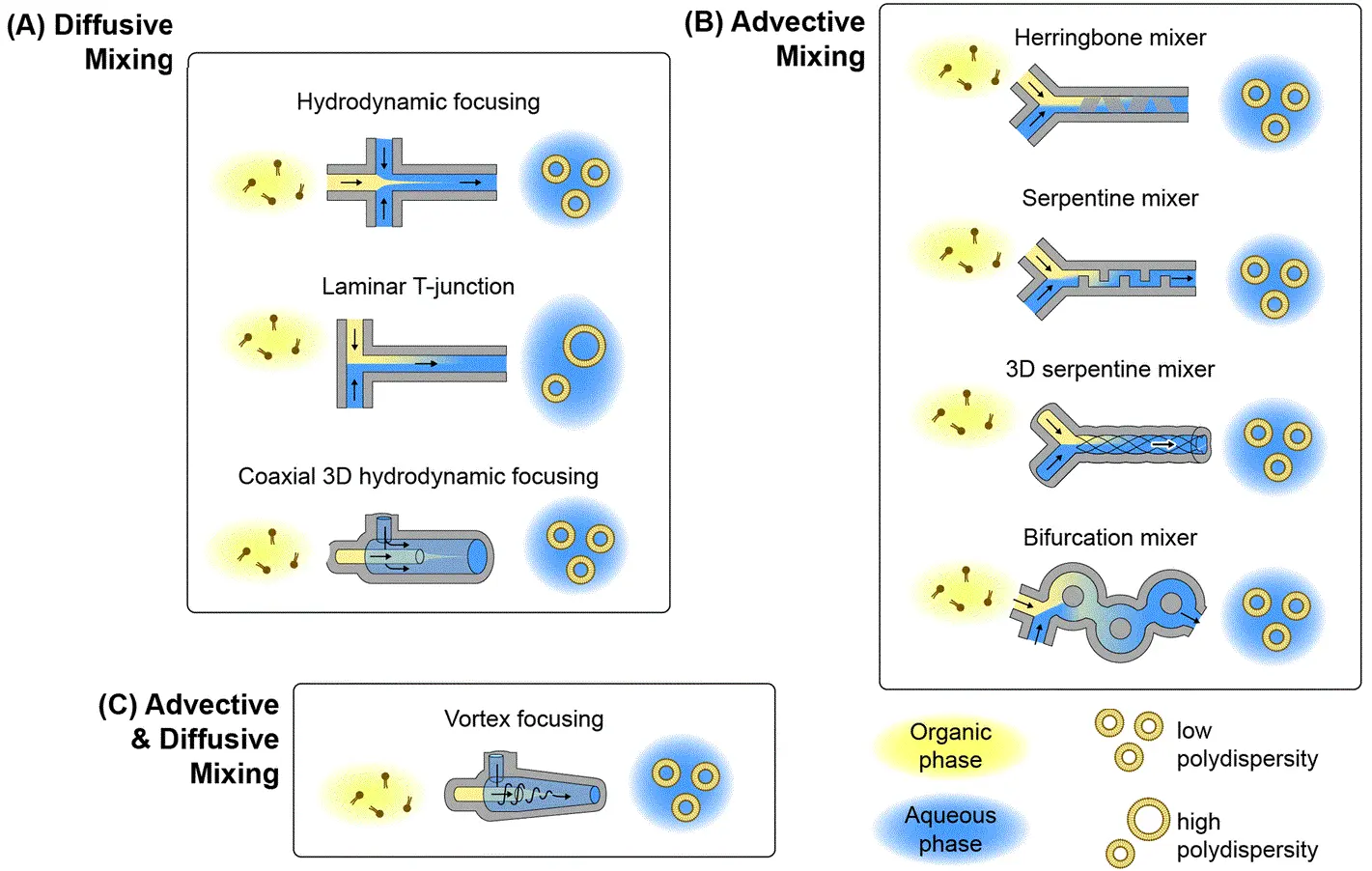
Figure 2: Microfluidic lipid nanoparticle production techniques
(From Mehraji, S. et al.; Lab Chip 2024, 24 (5), 1154–1174).
Explore more case studies of microfluidic techniques in LNP production:
2- Microfluidic Synthesis of Polymeric Nanoparticles:
Polymeric nanoparticles (PNPs) are increasingly used in drug delivery due to their versatile structure and the ability to encapsulate a wide range of therapeutic agents. These include hydrophilic and hydrophobic molecules, nucleic acids, and proteins. Unlike lipid-based nanoparticles, which are primarily lipid-based self-assemblies, PNPs are composed of polymeric materials such as poly(lactic-co-glycolic acid) (PLGA), polycaprolactone (PCL), and other biodegradable or biocompatible polymers. These polymers can form either matrix-type nanospheres or core-shell nanocapsules, providing flexibility in encapsulating different bioactive substances.9,15
The microfluidic synthesis of PNPs shares similarities with LNP production in the areas of precise control over particle size, size distribution, and encapsulation efficiency (Figure 3). Techniques for PNP synthesis include hydrodynamic focusing, nanoprecipitation, and coaxial flow systems, all of which are also employed in LNP synthesis. The primary difference lies in the material composition and the solvent systems used. 2,5,15,16
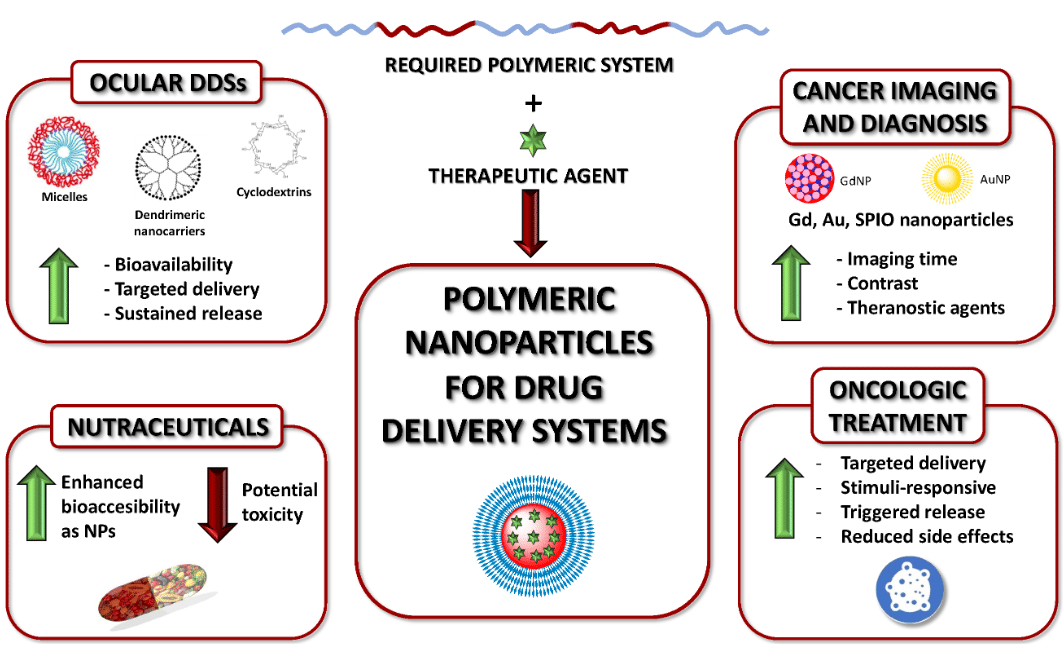
Figure 3: Polymeric nanoparticles for drug delivery
(From Begines, B. et al.; Nanomaterials 2020, 10 (7), 1403).
3- Microfluidic Production of Droplet-Based Microparticles
Microparticles made from biopolymers are emerging as important platforms for sustained drug delivery, cell therapy, and biomedical implants. Traditional batch production methods often suffer from poor size control and low reproducibility. Microfluidics enables the generation of uniform microparticles with precisely engineered properties, ensuring high-quality drug delivery systems.
This technique involves the generation of droplets within microchannels, where polymer solutions are encapsulated in immiscible carrier fluids. These droplets are then solidified through processes such as crosslinking, solvent evaporation, or polymerization. The geometry of the microchannels and the flow conditions can be finely tuned to control droplet size and formation frequency.5,9,17–19
These methods offer precision, reproducibility, and compatibility with various drug types and materials, making them an attractive choice for next-generation therapeutic systems.
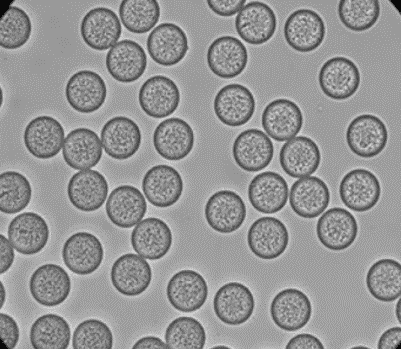
Figure 4: PLGA Microbeads encapsulating lysozyme (Produced by Secoya Technologies).
Table 1: Examples of drug encapsulation using microfluidics (Adapted from Parra Saldivar et al.; Front Biosci 2018, 10 (1), 74–91).
Material Used | Geometry | Carrier Material | Drug | Application | Ref. |
---|---|---|---|---|---|
Glass | Co-flow | Human serum albumin, poly(lactic acid) | Doxorubicin | Hepatic cancer | 20 |
PEEK and silica tube | T-junction | Poly(methyl acrylate), poly(acrylamide) | Ketoprofen, ranitidine | Suppression of gastric irritation effects | 21 |
Silicon | Flow focusing | PLGA | Ciclosporin | Immunosuppressive therapy | 22 |
PDMS | Co-flow with herringbone shape | Liposomes | Propofol | Anesthetic agent | 23 |
Quartz Chip | Flow-focusing | Hyaluronic acid, ethylenediamine | Dexamethasone | Cell differentiation of mesenchymal stem cells | 24 |
Glass | Co-flow and flow-focusing | Polycaprolactone, poly(vinyl alcohol), poly(ethylene glycol) | Bovine serum albumin | Protein therapy | 25 |
PDMS | T-junction | Poly(ethylene glycol) diacrylate | 5-fluorouracil | Cancer therapy | 26 |
PMMA | V-junction | Poly(methylsilsesquioxane) | Itraconazole | Antifungal drug for infections | 27 |
Explore more applications on droplet-based microfluidics:
- Microfluidic Application Notes
1-10 microns PLGA microsphere production using the RayDrop
Read more - Microfluidic Application Notes
Alginate Microcapsule Synthesis
Read more
Free content for better understanding on droplet production methods
Based Microneedle Systems for Carrier-Free Drug Delivery
Microfluidic technology enables the advancement of carrier-free drug delivery systems. By leveraging the precision and control provided by microfluidic platforms, drugs can be delivered directly to target sites, minimizing the need for conventional drug carriers. This results in a more efficient delivery system with better bioavailability and targeted release. Among the most promising components in this approach are micro-needles, which are highly integrated into microfluidic devices to optimize the delivery of therapeutics.2,28,29
Micro-Needles (MNs): These are devices that utilize arrays of microsized-needles, small, precise structures designed to penetrate the skin or other tissues for targeted drug delivery. These microneedles enable painless, minimally invasive drug administration, bypassing the need for traditional injections and oral delivery systems. The control offered by microfluidics ensures localized and controlled release of the drug, which can enhance the bioavailability and targeted action of therapeutics, particularly those that may degrade in the digestive system.
Types of Micro-Needles:
- Solid Micro-Needles: These are designed to create micro-channels in the skin, after which drugs can be applied topically, allowing them to diffuse passively through the skin. Solid MNs are typically made from materials such as (Silicon, Metals, Polymers).30
- Dissolving Micro-Needles: Composed of biodegradable materials, these MNs dissolve upon insertion into the skin, releasing the drug directly into the targeted tissue. Materials used for dissolving MNs include (Polyvinyl Alcohol (PVA), Polyvinylpyrrolidone (PVP), Polylactic Acid (PLA)).31
- Hydrogel Micro-Needles: These are made from swelling polymer materials and release drugs via the expansion of the hydrogel upon insertion into the skin. Materials for hydrogel MNs include (Polyethylene Glycol (PEG), Polyacrylamide (PAAm), Chitosan).32
- Hollow Micro-Needles: These are designed with a hollow core that allows for the direct infusion of drugs into the body through the needle. Hollow MNs are typically made from materials such as (Glass, Silicon, Metals, Polymers).33,34
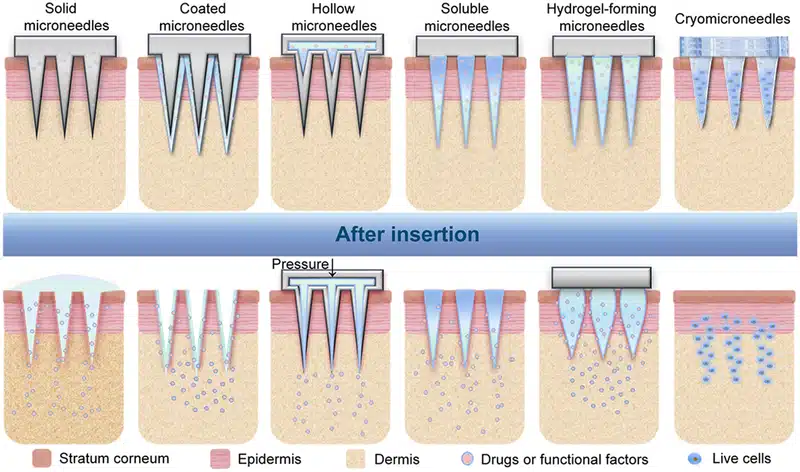
Figure 5: Types of microneedles and their corresponding drug delivery mechanisms
(From Zhang, Y. er al.; Exploration 2023, 3 (1), 20210170).
In Vitro Models for the Evaluation of Drug Delivery
To bridge the gap between conventional in vitro models and the complexity of human physiology, organ-on-a-chip (OOC) platforms have emerged as powerful tools in the evaluation of drug delivery systems. These models closely replicate the dynamic architecture and function of human tissues, offering insights into drug transport, absorption, and therapeutic response. As such, microfluidics in drug delivery has become an emerging technology for studying how nanocarriers interact with traverse biological barriers.35,36
Once a drug carrier enters the body, its journey to the target site is impeded by several key physiological barriers. Accurately modeling and understanding these obstacles is critical to the development of effective and safe drug delivery systems. Microfluidic OOC platforms offer a highly controlled environment where researchers can simulate and analyze these barriers in real time.
Key biological barriers include:
- Blood–Brain Barrier (BBB): This tightly regulated interface restricts the entry of most therapeutic agents into the brain. Microfluidic BBB-on-a-chip models co-culture endothelial cells, astrocytes, and pericytes under controlled shear flow to mimic the selective permeability and tight junctions characteristic of the human BBB. These systems are vital for evaluating brain-targeted drug delivery systems. 37
- Mucosal Diffusion Barrier: Present in areas such as the gastrointestinal, respiratory, and reproductive tracts, this consists of a dense mucus layer that traps and excludes foreign particles. Microfluidic models replicate mucus viscosity and secretion dynamics, allowing real-time observation of nanoparticle diffusion, penetration, and retention for oral and pulmonary drug delivery.38
- Cellular Permeability Barrier: Composed of epithelial or endothelial cell monolayers with tight junctions, this barrier controls both transcellular and paracellular transport. On-chip systems can simulate cellular architecture and mechanical stimuli, enabling studies of nanoparticle uptake, receptor-mediated transport, and barrier integrity modulation.39
- Biochemical Barrier: Enzymes and pH variations, particularly in the gut and lysosomal environments, can degrade or inactivate drugs before they reach their target. Microfluidic biochemical models simulate these conditions to test the stability and protective efficacy of nanocarriers under physiologically relevant stresses.2
The integration of these barriers into microfluidic organ-on-a-chip platforms enables more predictive and human-relevant preclinical evaluation.
Devices such as, (gut-on-a-chip, vessel-on-a-chip, blood–brain barrier-on-a-chip have demonstrated the potential of precision drug delivery using microfluidics.
As microfluidics in drug delivery continues to evolve, these platforms can be used in nanomedicine for evaluating pharmacokinetics, pharmacodynamics, and therapeutic index in a more human-relevant manner with reduced dependence on animal models.
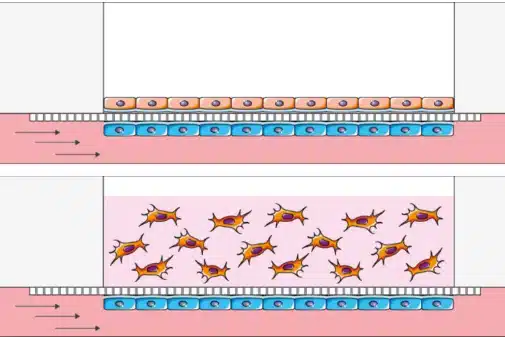
Figure 6: Creation of an endothelium-epithelium barrier for air liquid interface cell culture in 2D or 3D
(From BeOnChip).
An example in ocular drug delivery involves the application of microfluidic systems to replicate dynamic intraocular environments. By integrating flow control and temperature regulation, these platforms allow for the simulation of physiological conditions, such as intraocular pressure fluctuations and eye motion. This enables precise assessment of how these variables affect drug clearance and retention. The combination of automated sensing and synchronized flow across multiple in vitro models improves reproducibility and throughput in formulation screening, supporting more predictive and scalable testing for intraocular drug delivery.40
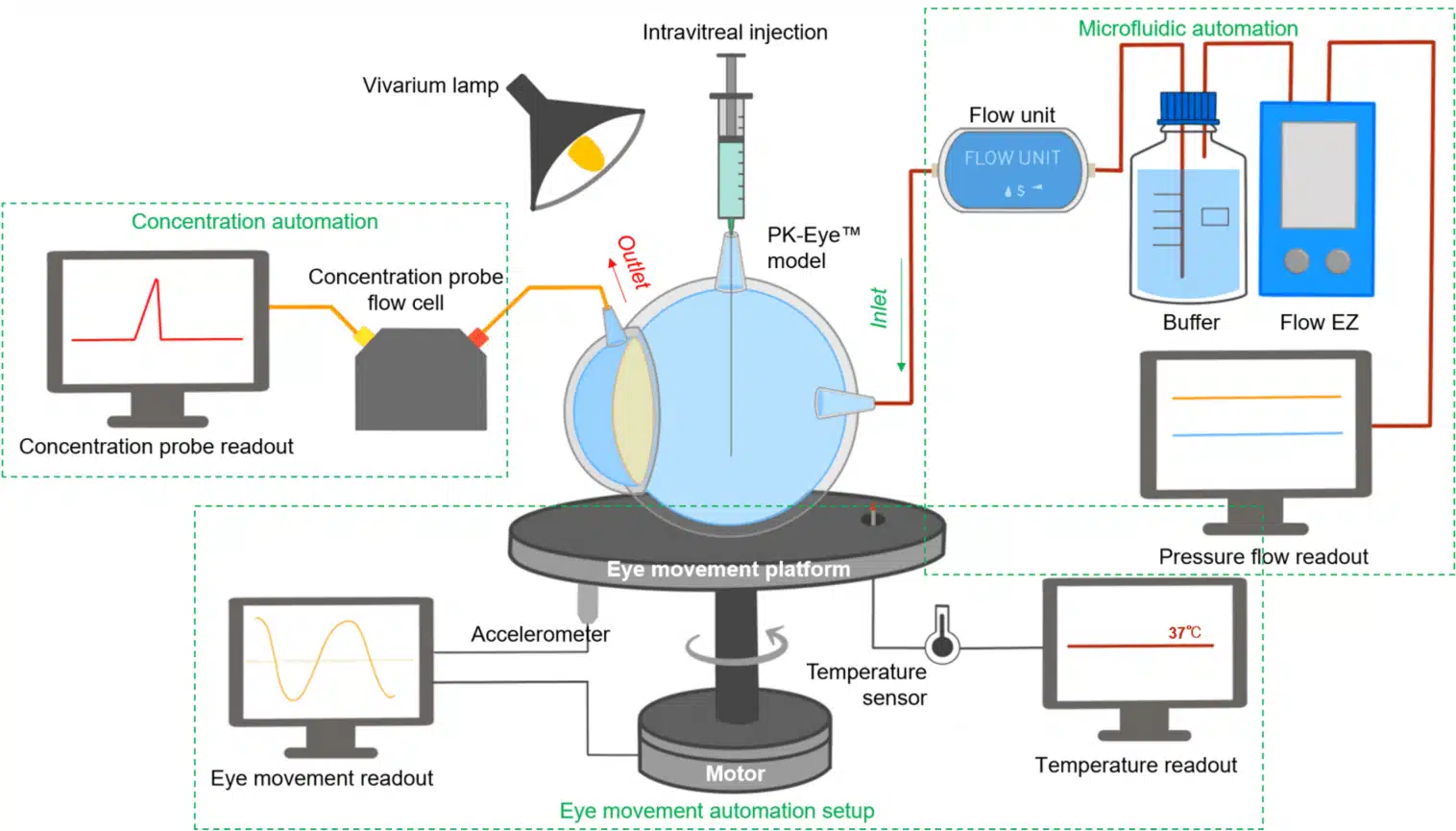
Figure 7: In vitro microfluidic monitoring platform for ocular drug delivery
(Awwad, S. el al.; Pharmaceutics 2023, 15 (5), 1444).
Microfluidics for Drug and Protein Crystallization in Pharmaceutical Research
Microfluidic systems are also transforming protein crystallization, a key process in drug development. Traditionally requiring large sample volumes, microfluidic platforms allow for the screening of crystallization conditions with minimal amounts of protein and reagents. By simulating various conditions like pH, temperature, and salt concentrations, these devices enable faster, more efficient crystallization.
Recent innovations, such as centrifuge-based microfluidic devices and semi-contact dispensing methods, have enhanced high-throughput screening and precision, reducing costs and accelerating drug development. This advancement plays a crucial role in creating high-quality protein crystals for structural analysis, aiding in more targeted drug delivery.41–43
An all-in-one platform for continuous generation of UV-cured core-shell microcapsules
Read MoreWebinar: Controlled UV-crosslinked microcapsule production using microfluidic technology
Read More
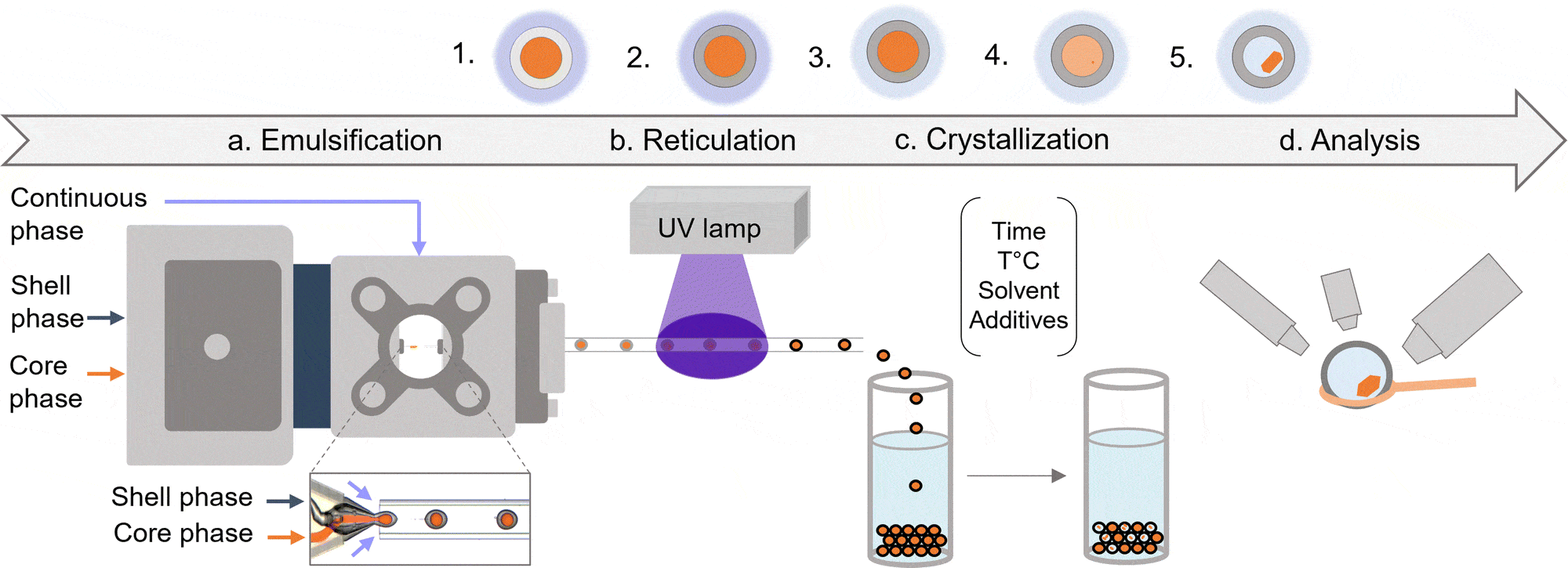
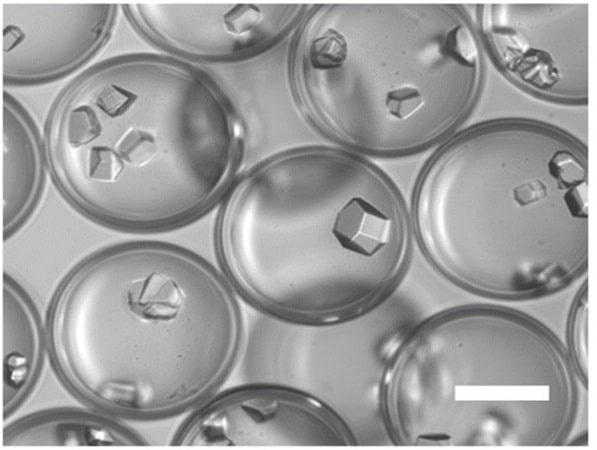
Figure 8: (Left)Schematic representation of an example of crystallization process from initial droplets. (Right) Lysozymes crystals inside microcapsules
(From Mettler, M. et al.; Chem. Commun. 59, 12739–12742 (2023)).
Conclusion
In this review, we highlighted the advancements in drug delivery systems enabled by microfluidics, which enhance bioavailability, drug efficiency, and nanoparticle performance. Despite progress, challenges remain in scaling these systems for clinical use, with the need for improved parallelization and simplified fabrication. Integrating microfluidics with organ-on-a-chip technology offers promising solutions for more accurate preclinical testing and personalized medicine. With continued interdisciplinary collaboration, microfluidics has the potential to transform further drug delivery and therapeutic applications.
👉 Ready to improve your drug delivery workflows? Contact our experts or explore our microfluidic pressure control systems.
Related Products
Expertises and resources
-
Expert Reviews: Basics of Microfluidics 10 Tips for Reliable Droplet Generation Read more
-
Microfluidics case studies Drug-Loaded Liposome Preparation Using Microfluidics Read more
-
Microfluidics case studies Real-Time Monitoring Platform for Ocular Drug Delivery, Integrating Fluigent’s Flow EZ Read more
-
Microfluidic Application Notes 1-10 microns PLGA microsphere production using the RayDrop Read more
-
Microfluidics Article Reviews Solid lipid nanoparticles for biologics and drug encapsulation Read more
-
Microfluidic Application Notes Alginate Microcapsule Synthesis Read more
-
Microfluidic Application Notes Encapsulation of multiple emulsions in a single droplet Read more
-
Microfluidics Article Reviews Human Blood Brain Barrier (BBB) permeability -on-chip assessment Read more
-
Microfluidics White Papers Microfluidic white paper – A review of Organ on Chip Technology Read more
-
Microfluidics Article Reviews Microfluidic technology for engineered nanoparticles in nanomedicine Read more
-
Microfluidic Application Notes Development of a human gut-on-chip to assess the effect of shear stress on intestinal functions Read more
-
Microfluidic Application Notes PLGA microcapsules synthesis Read more
-
Microfluidics White Papers Droplet-based Microfluidics Read more
-
Microfluidic Application Notes Liposome Nanoparticle Synthesis Read more
-
Expert Reviews: Basics of Microfluidics Microfluidic Flow Control: Comparison between peristaltic, syringe and pressure pumps for microfluidic applications Read more
References
1. Park, K. Controlled drug delivery systems: Past forward and future back. J. Controlled Release 190, 3–8 (2014).
2. Ma, Z., Li, B., Peng, J. & Gao, D. Recent Development of Drug Delivery Systems through Microfluidics: From Synthesis to Evaluation. Pharmaceutics 14, 434 (2022).
3. Li, C. et al. Recent progress in drug delivery. Acta Pharm. Sin. B 9, 1145–1162 (2019).
4. Jaradat, E., Weaver, E., Meziane, A. & Lamprou, D. A. Microfluidics Technology for the Design and Formulation of Nanomedicines. Nanomaterials 11, 3440 (2021).
5. Parra Saldivar, R. Microfluidics technology for drug delivery A review. Front. Biosci. 10, 74–91 (2018).
6. Zhang, H. et al. Microfluidics for nano-drug delivery systems: From fundamentals to industrialization. Acta Pharm. Sin. B 13, 3277–3299 (2023).
7. Mehraji, S. & DeVoe, D. L. Microfluidic synthesis of lipid-based nanoparticles for drug delivery: recent advances and opportunities. Lab. Chip 24, 1154–1174 (2024).
8. Jahn, A., Vreeland, W. N., Gaitan, M. & Locascio, L. E. Controlled Vesicle Self-Assembly in Microfluidic Channels with Hydrodynamic Focusing. J. Am. Chem. Soc. 126, 2674–2675 (2004).
9. Jahn, A. et al. Preparation of nanoparticles by continuous-flow microfluidics. J. Nanoparticle Res. 10, 925–934 (2008).
10. Suh, Y. K. & Kang, S. A Review on Mixing in Microfluidics. Micromachines 1, 82–111 (2010).
11. Nguyen, N.-T. & Wu, Z. Micromixers—a review. J. Micromechanics Microengineering 15, R1–R16 (2005).
12. Saorin, A. et al. Microfluidic production of amiodarone loaded nanoparticles and application in drug repositioning in ovarian cancer. Sci. Rep. 14, 6280 (2024).
13. Markwalter, C. E. & Prud’homme, R. K. Design of a Small-Scale Multi-Inlet Vortex Mixer for Scalable Nanoparticle Production and Application to the Encapsulation of Biologics by Inverse Flash NanoPrecipitation. J. Pharm. Sci. 107, 2465–2471 (2018).
14. Liu, Y., Cheng, C., Liu, Y., Prud’homme, R. K. & Fox, R. O. Mixing in a multi-inlet vortex mixer (MIVM) for flash nano-precipitation. Chem. Eng. Sci. 63, 2829–2842 (2008).
15. Leung, M. H. M. & Shen, A. Q. Microfluidic Assisted Nanoprecipitation of PLGA Nanoparticles for Curcumin Delivery to Leukemia Jurkat Cells. Langmuir 34, 3961–3970 (2018).
16. Begines, B. et al. Polymeric Nanoparticles for Drug Delivery: Recent Developments and Future Prospects. Nanomaterials 10, 1403 (2020).
17. Onan, D. et al. Microfluidics Based Particle and Droplet Generation for Gene and Drug Delivery Approaches. J. Biomed. Mater. Res. B Appl. Biomater. 113, e35530 (2025).
18. Hallan, S. S., Kaur, P., Kaur, V., Mishra, N. & Vaidya, B. Lipid polymer hybrid as emerging tool in nanocarriers for oral drug delivery. Artif. Cells Nanomedicine Biotechnol. 44, 334–349 (2016).
19. Mukherjee, A. et al. Lipid–polymer hybrid nanoparticles as a next-generation drug delivery platform: state of the art, emerging technologies, and perspectives. Int. J. Nanomedicine Volume 14, 1937–1952 (2019).
20. Kim, M.-K., Kim, M. A., Jenjob, R., Lee, D.-H. & Yang, S.-G. Capillary microfluidics-derived doxorubicin-containing human serum albumin microbeads for transarterial chemoembolization of hepatic cancer. Mater. Sci. Eng. C 62, 391–397 (2016).
21. Khan, I. U. et al. Microfluidic conceived pH sensitive core–shell particles for dual drug delivery. Int. J. Pharm. 478, 78–87 (2015).
22. Keohane, K., Brennan, D., Galvin, P. & Griffin, B. T. Silicon microfluidic flow focusing devices for the production of size-controlled PLGA based drug loaded microparticles. Int. J. Pharm. 467, 60–69 (2014).
23. Kastner, E., Verma, V., Lowry, D. & Perrie, Y. Microfluidic-controlled manufacture of liposomes for the solubilisation of a poorly water soluble drug. Int. J. Pharm. 485, 122–130 (2015).
24. Agnello, S. et al. Microfluidic production of hyaluronic acid derivative microfibers to control drug release. Mater. Lett. 182, 309–313 (2016).
25. Pessi, J. et al. Microfluidics-assisted engineering of polymeric microcapsules with high encapsulation efficiency for protein drug delivery. Int. J. Pharm. 472, 82–87 (2014).
26. Xue, P., Wu, Y., Menon, N. V. & Kang, Y. Microfluidic synthesis of monodisperse PEGDA microbeads for sustained release of 5-fluorouracil. Microfluid. Nanofluidics 18, 333–342 (2015).
27. Kucuk, I., Ahmad, Z., Edirisinghe, M. & Orlu-Gul, M. Utilization of microfluidic V-junction device to prepare surface itraconazole adsorbed nanospheres. Int. J. Pharm. 472, 339–346 (2014).
28. Riahi, R. et al. Microfluidics for advanced drug delivery systems. Curr. Opin. Chem. Eng. 7, 101–112 (2015).
29. Bilal, M. et al. Microneedles in Smart Drug Delivery. Adv. Wound Care 10, 204–219 (2021).
30. Pradeep Narayanan, S. & Raghavan, S. Solid silicon microneedles for drug delivery applications. Int. J. Adv. Manuf. Technol. 93, 407–422 (2017).
31. Ahmad, N. N., Ghazali, N. N. N. & Wong, Y. H. Concept Design of Transdermal Microneedles for Diagnosis and Drug Delivery: A Review. Adv. Eng. Mater. 23, 2100503 (2021).
32. Chen, S. et al. Microneedle‐Array Patch Fabricated with Enzyme‐Free Polymeric Components Capable of On‐Demand Insulin Delivery. Adv. Funct. Mater. 29, 1807369 (2019).
33. Trautmann, A., Roth, G.-L., Nujiqi, B., Walther, T. & Hellmann, R. Towards a versatile point-of-care system combining femtosecond laser generated microfluidic channels and direct laser written microneedle arrays. Microsyst. Nanoeng. 5, 6 (2019).
34. Zhang, Y. et al. Microneedle system for tissue engineering and regenerative medicine. Exploration 3, 20210170 (2023).
35. Bhatia, S. N. & Ingber, D. E. Microfluidic organs-on-chips. Nat. Biotechnol. 32, 760–772 (2014).
36. Van Der Helm, M. W. et al. Direct quantification of transendothelial electrical resistance in organs-on-chips. Biosens. Bioelectron. 85, 924–929 (2016).
37. Begley, D. J. Delivery of therapeutic agents to the central nervous system: the problems and the possibilities. Pharmacol. Ther. 104, 29–45 (2004).
38. Jia, Z., Guo, Z., Yang, C.-T., Prestidge, C. & Thierry, B. “Mucus-on-Chip”: A new tool to study the dynamic penetration of nanoparticulate drug carriers into mucus. Int. J. Pharm. 598, 120391 (2021).
39. Waheed, S. et al. Engineering nano-drug biointerface to overcome biological barriers toward precision drug delivery. J. Nanobiotechnology 20, 395 (2022).
40. Awwad, S. et al. Real-Time Monitoring Platform for Ocular Drug Delivery. Pharmaceutics 15, 1444 (2023).
41. Mettler, M., Dewandre, A., Tumanov, N., Wouters, J. & Septavaux, J. Single crystal formation in core–shell capsules. Chem. Commun. 59, 12739–12742 (2023).
42. Wang, L. et al. A centrifugal microfluidic device for screening protein crystallization conditions by vapor diffusion. Sens. Actuators B Chem. 219, 105–111 (2015).
43. Zhu, Y. et al. Nanoliter-Scale Protein Crystallization and Screening with a Microfluidic Droplet Robot. Sci. Rep. 4, 5046 (2014).