Giant Unilamellar Vesicles (GUVs) Production using Microfluidics
In this review, we propose an overview of the different techniques used to produce microsized aqueous compartments confined by a single lipid bilayer that separates them from the surrounding aqueous environment. These structures, called Giant Unilamellar Vesicles (GUVs) have become an important and versatile tool in biology but their production remains challenging.
After presenting the main batch methods and their limitations, we focus on the use of microfluidics as a promising tool for improving GUV production.
This review was written in collaboration with Secoya Technologies
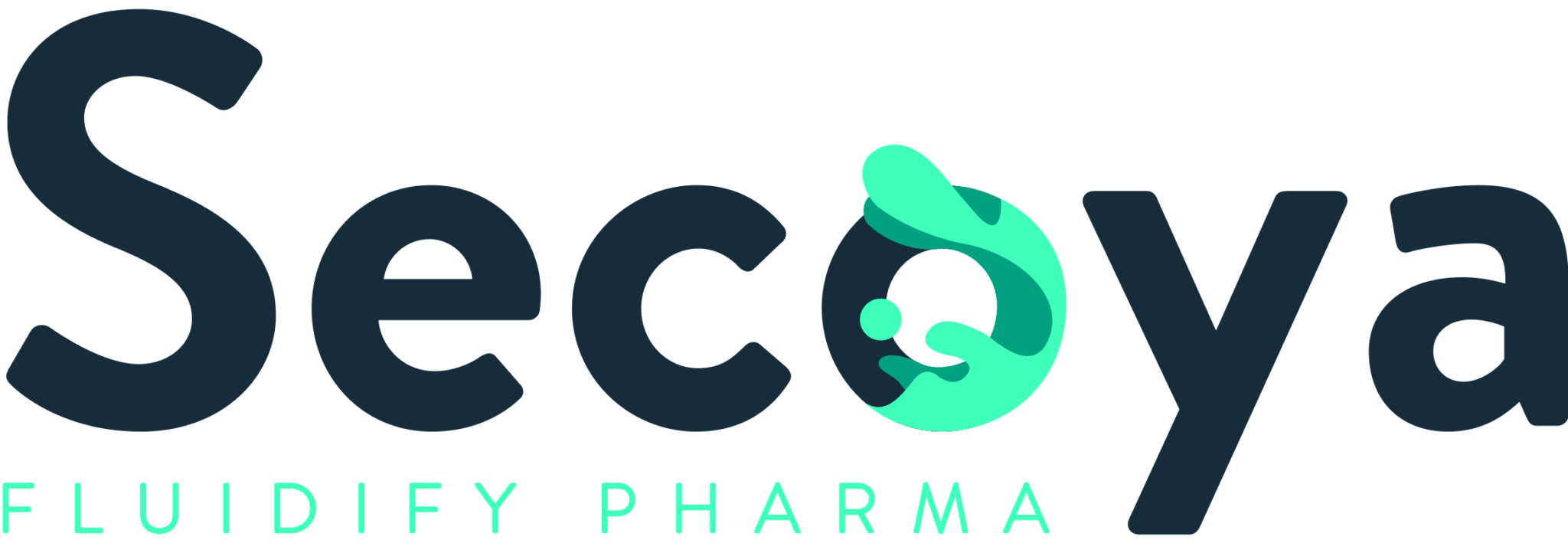
What are Giant Unilamellar Vesicles (GUVs)?
A liposome is a small artificial vesicle having a spherical shape that is formed by at least one lipid bilayer (which consists of two layers of lipid molecules. Its hydrophilic heads face outward, while the hydrophobic tails face inward, creating a flexible barrier that separates the interior from the exterior environment).1,2
Liposomes have long been used in biomedical applications as carriers of molecules in the human or animal body. For example, liposomes formed by a lipid bilayer encapsulating an aqueous core can transport a lipophilic drug in the lipid bilayer, while hydrophilic molecules can be transported in the aqueous core (figure 1).3
More recently, interest has emerged in liposomes formed by a single lipid bilayer (Unilamellar Vesicles, UV), because they present a membrane structure and biochemical properties close to those of the cell. They can therefore be used to study the properties of membrane and transmembrane transport in the cell, as they represent a simpler model compared to the complexity of biological cell membranes.4
Microsized unilamellar vesicles or GUV for Giant Unilamellar Vesicles, are of particular interest because their size, similar to biological cells, gives them a clear advantage for direct microscope visualization of phenomena occurring at a single membrane level, such as membrane dynamics and transport.4
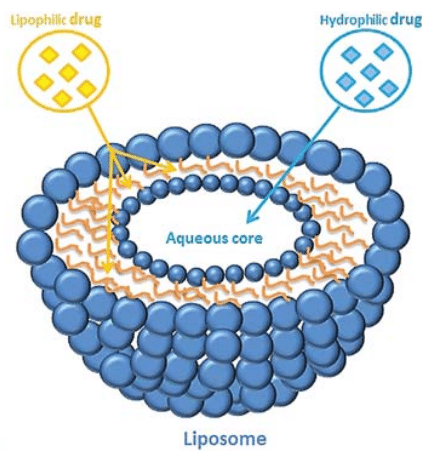
Figure 1: Depending on its affinity for water or lipid, the drug can be encapsulated either inside the aqueous core or inside the lipid bilayer.[3]
Traditional GUV Production Methods
While methods for producing nanometer-sized liposomes are well established in the field of healthcare as drug vectors, their use as engineered cells has been hampered by the lack of suitable methods for forming micrometer-sized vesicles.
Currently, the most common bulk methods for producing GUVs include gentle hydration, gel-assisted formation, and electroforming.5,6,7 However, these methods are slow, unreliable, and offer little control over the unilamellarity, size, and monodispersity of the vesicles. In addition, they do not allow high, uniform encapsulation of large and charged biomolecules. Another bulk method, known as the droplet transfer method, overcomes some of these drawbacks, while still presenting a severe limitation in terms of size control.
A. Gentle Hydration
In the conventional approach to forming Giant Unilamellar Vesicles, lipid films are created by dissolving lipids in chloroform at a concentration of approximately 1 mM inside a glass container.
The films are then dried with argon gas. Subsequent hydration using pure water or a buffer solution results in GUV formation after an incubation period at 25°C, typically lasting from a few hours to several days.
However, this method has drawbacks, including its slow pace due to extended incubation times, inefficiency resulting from poorly controlled lipid film swelling, and a broad size distribution of vesicles (ranging from a few micrometers to over 100 μm) with a lack of unilamellarity. Additionally, the formation of microsized unilamellar vesicles is limited to low ionic concentrations (up to 10 mM NaCl)3, 8, 9).
To address these challenges and enhance the approach, incorporating glass beads as a solid support for lipid coating can significantly improve the surface area for efficient lipid swelling and vesicle formation. This modification has been applied to various lipids, including both single-component lipids and mixed lipid systems.
While the method demonstrates good encapsulation efficiency under physiological lipid and buffer conditions, resulting vesicles remain polydisperse, and there is a lack of control over leaflet asymmetry (referring to the difference between the lipid composition and/or physical properties of the inner and outer layers of the lipid bilayer)10, 11.
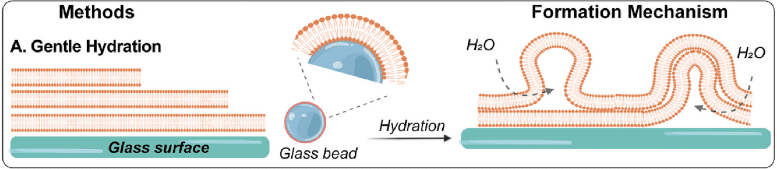
Figure 2: Gentle hydration: Hydration of lipid film coated on glass surfaces such as glass slides or glass beads.[10]
B. Electroformation
The Electroformation of Giant Unilamellar Vesicles method offers a solution to the sluggish swelling times observed in gentle hydration techniques. In this process, an alternating electric field is applied to a dry lipid film within a formation chamber containing two platinum electrodes, inducing the formation of surface-attached vesicles through hydration.
Alternatively, the lipid film can be deposited on glass slides coated with a conductive material, such as indium tin oxide, and separated by a spacer. This modification enables parallelization and high-throughput production of giant vesicles, making it a cost-effective approach.
Notably, the addition of an electrolyte in water, as opposed to water alone, can further expedite the electroformation process. However, it is crucial to avoid excessive concentrations of charged lipids in the mixture, as they may interfere with GUV production.
Despite its advantages, including the preparation of unilamellar vesicles using physiological lipids and buffers within specific parameters, electroformation faces challenges in controlling leaflet asymmetry and achieving optimal encapsulation efficiency10, 12, 13, 14.
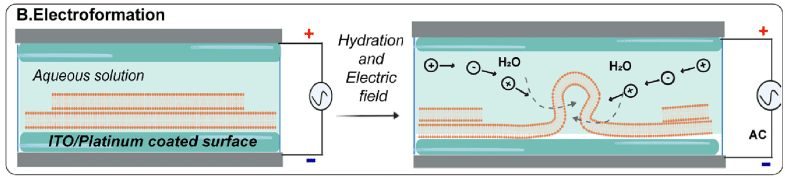
Figure 3: Electroformation – Lipid film coated on a conducting surface and hydrated in the presence of an electric field where vesicle formation occurs.[10]
C. Gel-assisted hydration
Gel-assisted hydration allows the production of GUVs at physiological ionic strength. A hydrogel-forming polymer is dried to deposit a lipid film on a glass surface. Then, the film and the hydrogel are hydrated with a buffer solution so that giant unilamellar vesicles are formed at the gel/water interface. The size of the vesicles produced could be raised by increasing the buffer ionic strength.
This method is faster than gentle hydration and electroformation and it can be used at physiological ionic strength as well as for different lipid compositions. Also, the risk of degrading the lipids faced by electroformation does not arise. By employing the gel-assisted hydration method, it is possible to create GUVs with specific lipid composition and buffer, allowing a degree of control over size and biomolecule encapsulation.
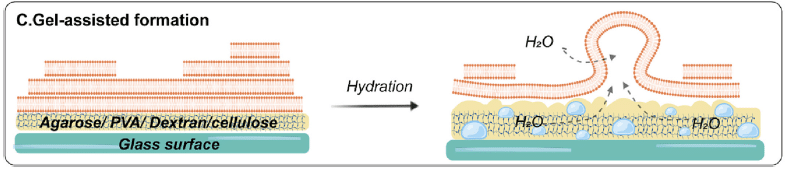
Figure 4: Gel-assisted formation: Hydration carried out on lipid-coated porous gel/fiber surfaces. [10]
D. Droplet transfer method
The droplet transfer method consists of introducing an aqueous buffer solution (forming the inner solution of giant vesicles) into an organic solution containing lipids. A water-in-oil (w/o) emulsion is formed by pipetting or vortexing. The emulsion is then placed in a microtube containing an aqueous buffer solution (forming the outer phase of the vesicles) and the lipids solution which will form the second lipid bilayer.
These two phases are immiscible and thus form two heterogeneous layers. By gravity, the w/o emulsions are transferred to the interface between the lipid and buffer solutions, thus forming the outer solution of the giant vesicles.
Consequently, the giant vesicles with the lipid bilayer are formed from the w/o emulsions. Compared to the gentle hydration method, this technique gives more monodispersed, unilamellar, and well-encapsulated giant vesicles but with oil remnants in the membrane, which can alter properties like viscosity. Also, this process allows only low incorporation of cholesterol due to its hydrophobic nature. The formation and stability of droplets plays a crucial role in the creation of giant unilamellar vesicles using this approach.
Therefore, controlling the size of these droplets allows for GUV size regulation, which is not attainable through the bulk water-in-oil (w/o) methods (pipetting/vortexing).17,18,19
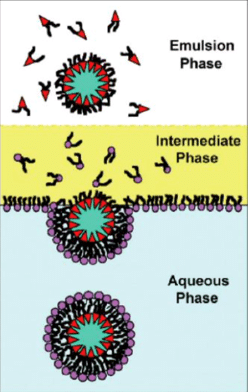
Figure 5 : Droplet transfer method. Water droplets in lipid-saturated oil stabilized by lipid molecules destined for the inner interface fall under their own weight in an intermediate phase of lipid-saturated oil heavier than the emulsion phase. Lipids form a monolayer at the oil/water interface and the bottom aqueous phase receives the formed vesicle. [5]
How does microfluidics enhance the GUV production process?
Microfluidics, through water-in-oil-in-water (W/O/W) double emulsions with lipids in the oil phase, emerged as a promising tool for GUV production compared to the traditional bulk methods and their drawbacks.
Typically, the production of giant unilamellar vesicles in microfluidics is a two-step process, as depicted in figure 6.
In the first step, an aqueous solution is pinched by an organic phase containing dissolved lipids. This results in the formation of water-in-oil (W/O) single emulsion droplets with a monolayer of lipids assembled at the interface. The single emulsion is then sheared into droplets by a second aqueous solution to form W/O/W double emulsions. Lipids can be present in the outer aqueous solution in the form of small vesicles and will form the second layer at the interface. The w/o/w method produces vesicles at high throughput, demonstrating effective size control and high encapsulation efficiency.
However, the presence of residual oil in the bilayer is a recurring problem when using these processes for vesicle production.20
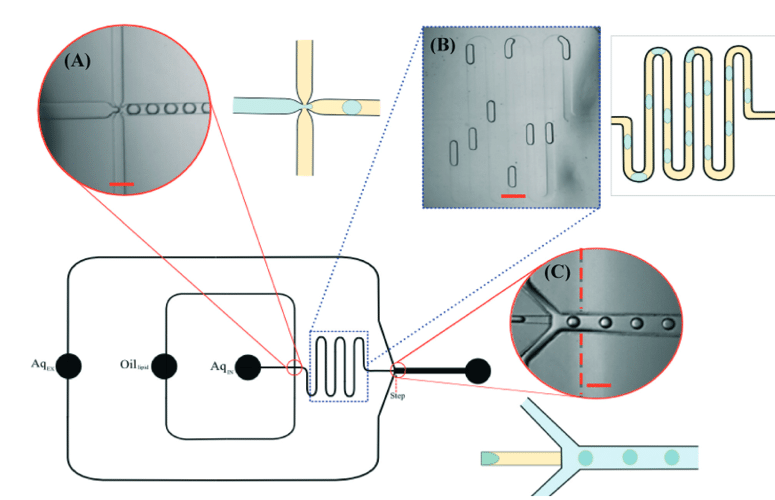
Figure 6: Schematic of vesicle production line using two successive flow-focusing junctions for double emulsion generation. A W/O droplet is formed at the first, hydrophobically coated junction while a double emulsion W/O/W is formed at the second, hydrophilic coated junction. [20]
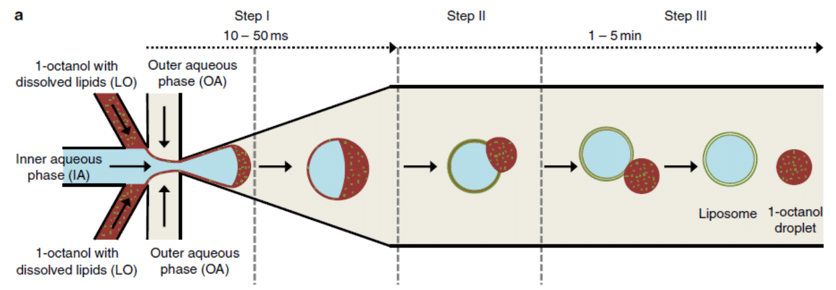
Figure 7 : Octanol separation from the formed GUV where the octanol is accumulated in front of the GUV in the direction of the flow before gradually being detached. [8]
Another option is to use a partially water-miscible oil, such as octanol, as the shell phase.8When octanol-containing lipids are extracted in water, lipids assemble along both interfaces to form giant unilamellar vesicles (figure 7).
This technique, first described by Deshpande et al. (2016), consists of forming double emulsion droplets from an aqueous core phase and an ultra-thin organic shell phase (lipid dissolved in 1-octanol) flowing through the outer aqueous phase (continuous phase). The droplets formed present an octanol pocket on one side of the droplet, in the direction of the flow. After a few minutes, oil-free vesicles are obtained after full detachment of the octanol pocket due to the dewetting. Next, the created vesicles and octanol droplets can be separated thanks to their density difference.
This microfluidic technique allows efficient encapsulation of biomolecules with precise size control of the vesicles. In addition, it presents the opportunity to automate the process and create a more complex arrangement of leaflets while enhancing control over the number and composition of each leaflet.
However, a question remains regarding the biomimetic properties of the lipid vesicles due to the usage of surfactants and additives in both the aqueous phases that can affect the biophysical membrane properties, hampering their use as cell mimics.9,10
Yandrapalli et al. succeeded in generating giant unilamellar vesicles without the use of additives or surfactants by using a well-designed PDMS-on-glass chip with a double flow focusing junction.21 This design enabled the generation of vesicles in various sizes, ranging from approximately 10 to 130 μm, using either neutral or charged lipids and under physiological buffer conditions.
Characterization tests confirmed the purity, functionality, and stability of these vesicle membranes through lipid diffusion, protein incorporation, and leakage assays.
Furthermore, their potential as artificial cells was demonstrated by increasing their complexity, such as encapsulating plasmids, smaller liposomes, mammalian cells, and microspheres.10,21
Overall, the use of microfluidics to produce microsized unilamellar vesicles overcomes most of the limitations encountered in batch processes. It allows a high level of control over size and unilamellarity, as well as a high production rate and high encapsulation efficiency.
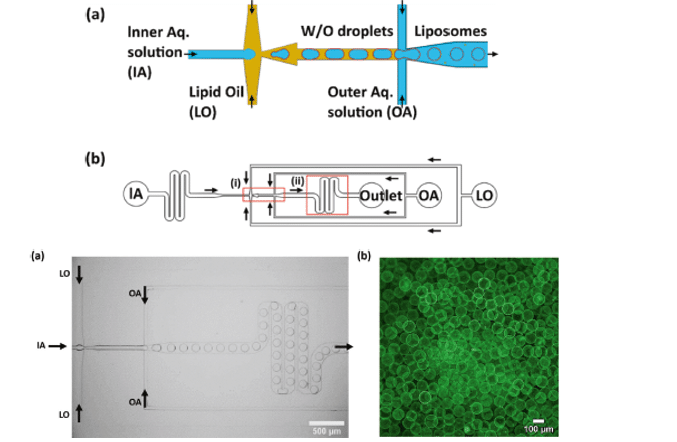
Figure 8: Microfluidic device design presented by Yandrapalli et al. and an example of confocal fluorescence image of GUVs [21]
GUV Applications
The applications of giant vesicles can be categorized into two main areas: understanding membrane biophysical processes and the construction of synthetic cells.
In the field of membrane biophysics, giant vesicles have been used to study phenomena such as membrane phase separation, fluid/gel-like domains, and the effects of proteins and specific lipids on membrane properties. These studies have led to insights into various biological processes, including fission, fusion, and shape changes in cells.
Additionally, researchers have explored the interaction of antimicrobial peptides and the transport properties of membrane proteins in giant vesicles.10,22,23,24
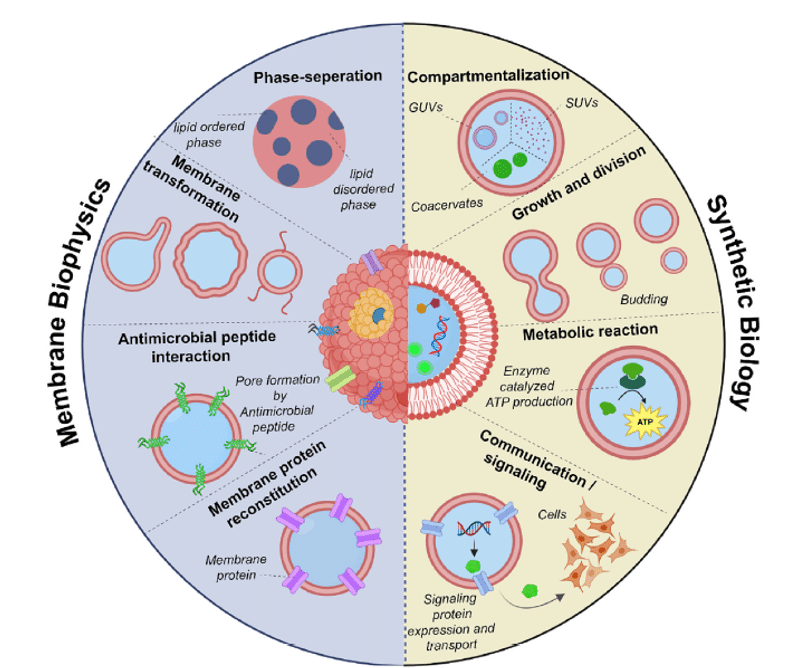
Figure 9: Application of Giant Unilamellar Vesicles.[10]
In the context of building synthetic cells, giant vesicles have served as an initial component. They have been used to mimic cellular features such as compartmentalization, communication, motility, growth, and reproduction. This includes the creation of vesicle-in-vesicle structures resembling cell organelles and the development of systems that enable enzymatic reactions and regulated transcription.
Controlled growth, budding, and division of giant vesicles have been achieved through various external and internal stimuli.
Furthermore, synthetic cells constructed from giant vesicles have been engineered to communicate with natural cells and to perform functions such as replication of genetic material, self-reproduction, and self-organizing protein expression.10,25,26
In summary, creating GUVs for artificial cells involves two key goals: developing a complex and biologically analogous membrane while achieving a multicomponent lumen. These requirements ensure that GUVs accurately mimic the properties and functions of natural cells, making them suitable containers for artificial cells. Therefore, the fabrication method of giant unilamellar vesicles remains crucial.27
Giant vesicles have also found applications in drug delivery, including non-intravenous routes. The development of microfluidic-based high-throughput techniques has expanded the possibilities for encapsulating larger therapeutic molecules like DNA, RNA, and enzymes, making them suitable for engineering complex cargo delivery systems responsive to external triggers.28,29
Conclusion and perspective
In the past decade, there has been significant progress in the development of novel techniques for preparing giant unilamellar vesicles (GUVs). These advances are essential for understanding membrane biophysical processes and constructing synthetic cells. Various GUV production methods have their own advantages and drawbacks, with some providing better control over factors like lipid composition physiological conditions, leaflet arrangement, oil-free membranes, lamellarity, and encapsulation efficiency.
Film-hydration techniques, although easy to use, have lower encapsulation efficiency, making them less suitable for bottom-up biology applications. Other methods like water-oil emulsion and vesicles from lipid bilayer techniques offer better control over leaflet arrangement and biomolecule encapsulation. The use of microfluidic modules with emulsion techniques has allowed for precise control over GUV size and composition.
The long-term goal is to create hierarchical structures resembling multi-cellular organisms using GUVs, with applications in drug delivery and proto-tissue architectures. The ongoing development of high-yield preparation techniques will create new possibilities in GUV applications.
Properties of Giant unilamellar vesicles (GUV) | ||||||
Preparation Methods | Dispersity | Lamellarity | Leaflet Asymmetry | Oil content | Physiological lipid and buffer | Encapsulation |
Gentle Hydration | Polydisperse | Mutlilamellar | No | No | Yes | Moderate |
Gel-assisted hydration | Polydisperse | Unilamellar | No | No | Yes | Moderate |
Electroformation | Polydisperse | Unilamellar | No | No | Yes | Moderate |
Droplet transfer formation (bulk) | Polydisperse | Unilamellar | Yes | Yes | No | Efficient |
Microfluidics: double emulsion technique | Highly monodisperse | Unilamellar | No | Yes | Yes | Efficient |
Table 1: Summary of selected preparation methods of GUVs. Adapted from [10] and [27]
Learn more about GUV production techniques and their application in this paper review from the HARSHA BAJAJ LAB (CSIR- National Institute for Interdisciplinary Science and Technology, India).
Discover the Raydrop and its platform for double emulsions
Double emulsion microfluidic devices, although promising to produce Giant Unilamellar Vesicles, can be time-consuming to set up and, in the case of PDMS-based microfluidics require access to soft-lithography facilities. While laboratory-made glass capillary droplet generators can bypass the need for soft-lithography, this method involves relatively expensive and difficult-to-master capillary preparation technology.
In this context, the Raydrop developed by Secoya Technologies is an ideal device for the non-expert in microfluidics who wants to produce reproducible double emulsion quickly and easily.
The Raydrop relies on the use of couples of capillaries perfectly aligned in a metallic reservoir.
The first capillary is terminated with a 3D-printed nozzle and injects the droplet phases (core and shell) into the junction.
The second one, the collection capillary, is the only output of the system, so it collects the double emulsion carried by the continuous phase filling the reservoir under pressure.
The droplets are produced by the controlled squeezing of the core and shell phases by the continuous phase at the entrance of the collection capillary.
Thanks to the 3D axisymmetric geometry, the shell and core phases are never in contact with the wall of the device which prevents the need for any surface treatment to generate w/o/w and o/w/o double emulsion.
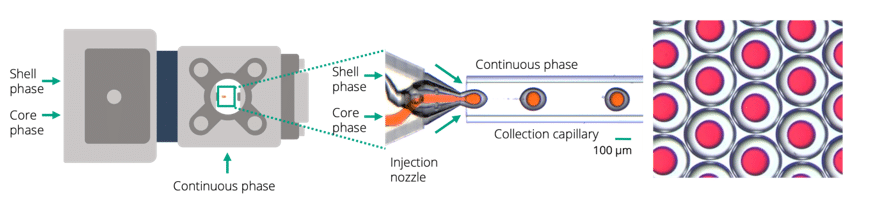
Figure 10: Raydrop for the production of core-shell double emulsion
The RayDrop is made of three metallic parts fully removable: two inserts on each side supporting capillaries and a central box with two glass windows for easy observation. The device is connected to fluid supplies and collection tubing by means of standard microfluidic tubing and nuts.
To make the Raydrop as easy to use as possible and minimize the microfluidics learning curve, Secoya, and Fluigent have developed a complex emulsion platform that includes all the equipment needed to operate the Raydrop: Fluigent pressure-based pumps and flow-meter, valves, filters, and a visualization module. Thanks to this platform, a non-expert user can master double emulsion production within a day and focus on what really matters for his or her research.
Related products
Related resources
- Microfluidics Case Studies
University of Cambridge: Microfluidic GUV production and testing
Read more - Microfluidic Application Notes
Liposome Nanoparticle Synthesis
Read more - Microfluidics Article Reviews
Microfluidic technology for engineered nanoparticles in nanomedicine
Read more - Expert Reviews: Basics of Microfluidics
Flow control for droplet generation using syringe pumps and pressure-based flow controllers
Read more - Microfluidics Article Reviews
A mRNA encapsulation platform integrating Fluigent’s FlowEZ
Read more - Microfluidic Application Notes
PLGA nanoparticle synthesis using 3D microfluidic hydrodynamic focusing
Read more
References
- Bangham AD, Horne RW. Negative staining of phospholipids and their structura lmodification by surface-active agents as observed in the electron microscope. J Mol Biol 1964;8. https://doi.org/10.1016/S0022-2836(64)80115-7. 660-IN10.
- Bangham AD, Standish MM, Watkins JC. Diffusion of univalent ions across thelamellae of swollen phospholipids. J Mol Biol 1965;13:238–52. https://doi.org/ 10.1016/S0022-2836(65)80093-6.
- Laouini, A.; Jaafar-Maalej, C.; Limayem-Blouza, I.; Sfar, S.; Charcosset, C.; Fessi, H. Preparation, Characterization and Applications of Liposomes: State of the Art. j coll sci biotechnol 2012, 1 (2), 147–168. https://doi.org/10.1166/jcsb.2012.1020.
- Weinberger, A.; Tsai, F.-C.; Koenderink, G. H.; Schmidt, T. F.; Itri, R.; Meier, W.; Schmatko, T.; Schröder, A.; Marques, C. Gel-Assisted Formation of Giant Unilamellar Vesicles. Biophysical Journal 2013, 105 (1), 154–164. https://doi.org/10.1016/j.bpj.2013.05.024.
- Reeves, J. P.; Dowben, R. M. Formation and Properties of Thin-Walled Phospholipid Vesicles. J. Cell. Physiol. 1969, 73 (1), 49–60. https://doi.org/10.1002/jcp.1040730108.
- Horger KS, Estes DJ, Capone R, Mayer M. Films of agarose enable rapid formation of giant liposomes in solutions of physiologic ionic strength. J Am Chem Soc, 2009; 131:1810–9. https://doi.org/10.1021/ja805625u.
- Witkowska, A.; Jablonski, L.; Jahn, R. A Convenient Protocol for Generating Giant Unilamellar Vesicles Containing SNARE Proteins Using Electroformation. Sci Rep 2018, 8 (1), 9422. https://doi.org/10.1038/s41598-018-27456-4.
- Deshpande, S.; Caspi, Y.; Meijering, A. E. C.; Dekker, C. Octanol-Assisted Liposome Assembly on Chip. Nat Commun 2016, 7 (1), 10447. https://doi.org/10.1038/ncomms10447
- Teh, S.-Y.; Khnouf, R.; Fan, H.; Lee, A. P. Stable, Biocompatible Lipid Vesicle Generation by Solvent Extraction-Based Droplet Microfluidics. Biomicrofluidics 2011, 5 (4), 044113. https://doi.org/10.1063/1.3665221.
- Nair, K. S.; Bajaj, H. Advances in Giant Unilamellar Vesicle Preparation Techniques and Applications. Advances in Colloid and Interface Science, 2023, 318, 102935. https://doi.org/10.1016/j.cis.2023.102935.
- Nourian Z, Roelofsen W, Danelon C. Triggered gene expression in fed-vesicle microreactors with a multifunctional membrane. Angew Chem Int Ed 2012;51: 3114–8. https://doi.org/10.1002/ANIE.201107123.
- Oropeza-Guzman E, Ri´os-Ramírez M, Ruiz-Su´arez JC. Leveraging the coffee ring effect for a defect-free electroformation of giant unilamellar vesicles. Langmuir 2019;35:16528–35. https://doi.org/10.1021/acs.langmuir.9b02488.
- Zhu C, Li Q, Dong M, Han X. Giant unilamellar vesicle microarrays for cell function study. Anal Chem 2018;90:14363–7. https://doi.org/10.1021/acs. analchem.8b03825
- Kang YJ, Wostein HS, Majd S, Kang YJ, Wostein HS, Majd S. A simple andversatile method for the formation of arrays of giant vesicles with controlled size and composition. Adv Mater 2013; 25:6834–8. https://doi.org/10.1002/ ADMA.201303290
- Mora NL, Hansen JS, Gao Y, Ronald AA, Kieltyka R, Malmstadt N, et al. Preparation of size tunable giant vesicles from cross-linked dextran(ethylene glycol) hydrogels. Chem Commun 2014; 50:1953–5. https://doi.org/10.1039/C3CC49144G
- Kresse KM, Xu M, Pazzi J, García-Ojeda M, Subramaniam AB. Novel application of cellulose paper as a platform for the macromolecular self-assembly ofbiomimetic giant liposomes. ACS Appl Mater Interfaces 2016; 8:32102–7. https://doi.org/10.1021/acsami.6b11960.
- Pautot, S.; Frisken, B. J.; Weitz, D. A. Engineering Asymmetric Vesicles. Proceedings of the National Academy of Sciences 2003, 100 (19), 10718–10721. https://doi.org/10.1073/pnas.1931005100.
- Elani Y, Purushothaman S, Booth PJ, Seddon JM, Brooks NJ, Law RV, et al. Measurements of the effect of membrane asymmetry on the mechanical properties of lipid bilayers. Chem Commun 2015;51:6976–9. https://doi.org/10.1039/C5CC00712G.
- Ip T, Li Q, Brooks N, Elani Y. Manufacture of multilayered artificial cell membranes through sequential bilayer deposition on emulsion templates. ChemBioChem 2021;22:2275–81. https://doi.org/10.1002/CBIC.202100072.
- Karamdad, K.; Law, R. V.; Seddon, J. M.; Brooks, N. J.; Ces, O. Preparation and Mechanical Characterisation of Giant Unilamellar Vesicles by a Microfluidic Method. Lab Chip 2014, 15 (2), 557–562. https://doi.org/10.1039/C4LC01277A
- Yandrapalli, N.; Petit, J.; Bäumchen, O.; Robinson, T. Surfactant-Free Production of Biomimetic Giant Unilamellar Vesicles Using PDMS-Based Microfluidics. Commun Chem 2021, 4 (1), 1–10. https://doi.org/10.1038/s42004-021-00530-1.
- Ramamurthi KS, Lecuyer S, Stone HA, Losick R. Geometric cue for protein localization in a bacterium. Science 2009;323:1354. https://doi.org/10.1126/SCIENCE.1169218.
- Litschel T, Ramm B, Maas R, Heymann M, Schwille P. Beating vesicles: encapsulated protein oscillations cause dynamic membrane deformations. Angew Chem Int Ed 2018;57:16286–90. https://doi.org/10.1002/anie.201808750.
- Ganzinger KA, Merino-Salom´on A, García-Soriano DA, Butterfield AN, Litschel T, Siedler F, et al. FtsZ reorganisation facilitates deformation of giant vesicles in microfluidic traps. Angew Chem Int Ed2020. https://doi.org/10.1002/anie.202001928. anie.202001928.