Mimicking tumor microenvironment using a 3D microfluidic model to improve cancer investigations
An article published on Lab on a Chip 2024 results from a collaboration between the Department of Chemical Engineering (Delft University of Technology) and the Department of Cell and Chemical Biology Oncode Institute (Leiden University of Medical Center) in the Netherlands.
The paper presents a new model using microfluidics to study lung cancer. It mimics the tumor microenvironment (TME) including the extracellular matrix (ECM) and biophysical forces such as interstitial flow (IF). Results showed that considering biophysical forces and cytokines is crucial in cancer investigations. A precise flow control by Fluigent’s MFCS series mimics interstitial flow. Thisproves that the model potentiates TGF-β in cancer cells by Smad pathway promoting cellular invasion.
A Paper from the Delft University of Technology in The Netherlands
Rahman, Z. et al. Interstitial flow potentiates TGF-β/Smad-signaling activity in lung cancer spheroids in a 3D-microfluidic chip. Lab Chip 24, 422–433 (2024). https://doi.org/10.1039/D3LC00886J
This article, published in Lab on a Chip 2024, results from a collaboration between the Department of Chemical Engineering (Delft University of Technology) and the Department of Cell and Chemical Biology Oncode Institute (Leiden University of Medical Center) in The Netherlands.
The Department of Chemical Engineering (Delft University of Technology) is divided into several research sections, all of which are based on one objective: being the leader of science involving all fields (chemistry, engineering, molecule, and device design). Among these sections, “Product and Process Engineering” includes the science of organ-on-a-chip studies. Dr. Pouyan Boukany is one of the principal investigators of this section, he works in several fields including tumor microenvironment at the interface of microfluidics and biology to understand fundamental issues.
The study demonstrated the crucial role of tumor microenvironment in cancer investigations using a 3D microfluidic model.
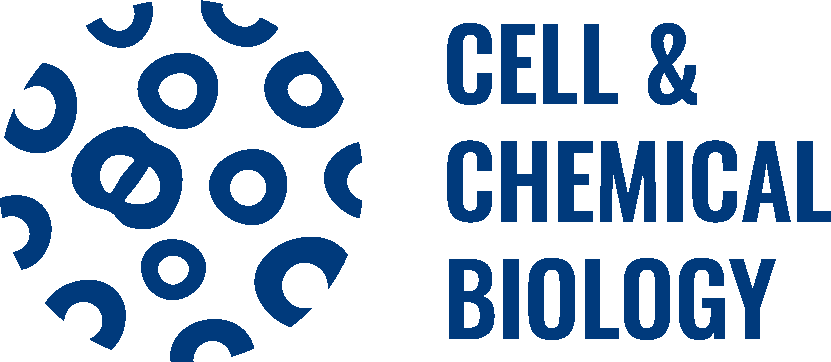
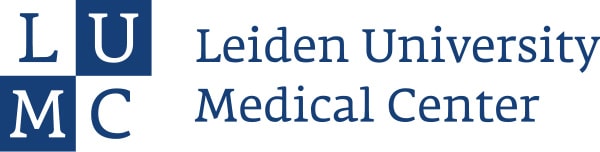
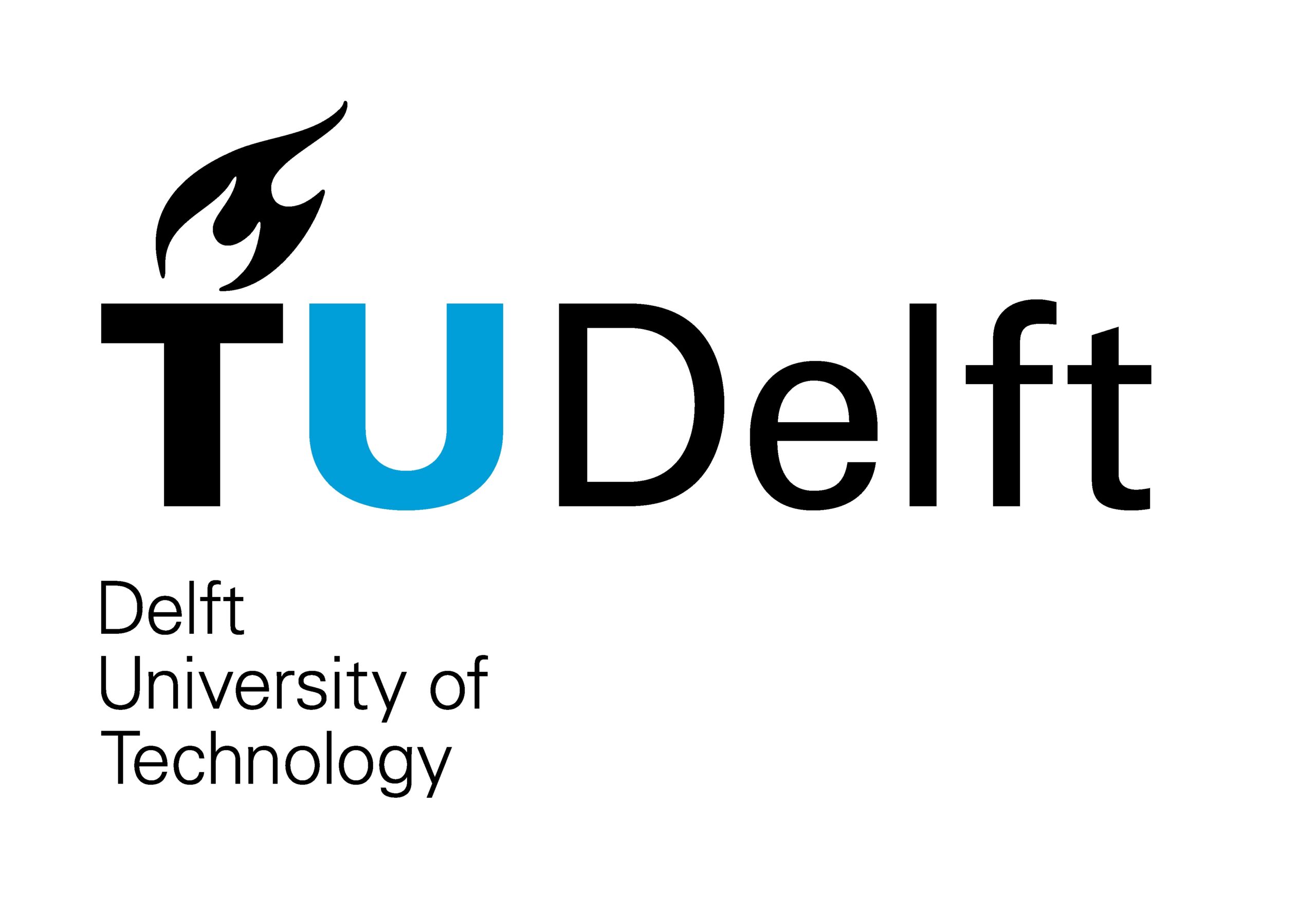
The crucial role of the tumor microenvironment (TME)
What is a tumor microenvironment?
Within an organism, cells interact with each other and with the extracellular matrix to adapt their progression according to the signals they receive. In the case of a tumor, cells do the same in an environment called tumor microenvironment that promotes their proliferation, migration, and invasion. TME provides tumor cells with several protections, including evasion of the immune system and apoptosis to increase cancer cell propagation (Figure 1). 1,2
How does TME influence cancer cell behavior
Within TME, cancer mechanosensitive cells can capture changes and must react to these changes. They use mechanotransduction to transform a modification of biophysical forces at the cell’s surface, such as the interstitial flow (IF), into a biochemical signal. Because of shear, compressive, and tensional forces, IF remodels the extracellular matrix and triggers signaling pathways such as Yap/Taz and a biochemical response by cytokines (TGF-β) production.
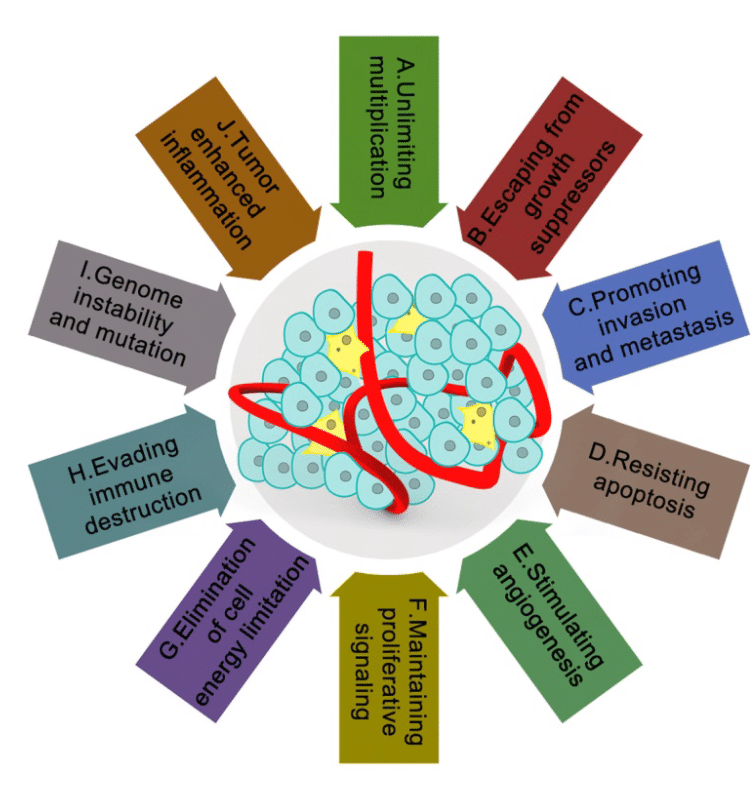
Figure 1: The tumor microenvironment and their characteristics in cancer2
Introduction of signaling pathways
Yap/Taz pathway in cancer mechanotransduction
In response to changes that involve mechanotransduction, different pathways can be activated as Yap/Taz, which is well-known in this process (Figure 2).3 Yap/Taz is only effective when the HIPPO pathway is inactive. Indeed, the HIPPO pathway can be inactive or active, and their cell consequences are opposite. In the case of inactive HIPPO, Yap/Taz is functional and can bind with Tead, a transcription factor, to activate its target genes (Figure 3).4 Among them, CTGF is present, which is involved in the regulation of proteins such as vimentin. The upregulation of this intermediate filament protein is known for its poor prognosis in cancer and its involvement in tumoral progression.5
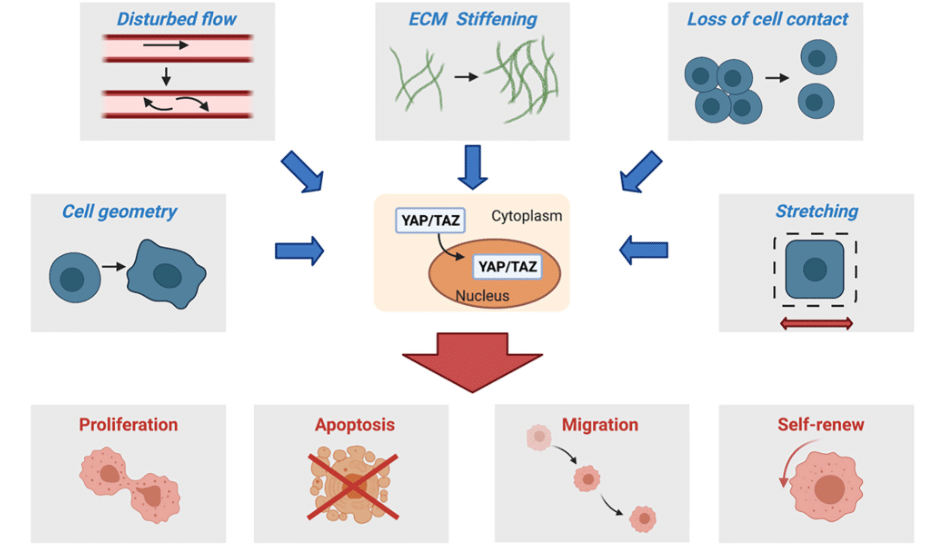
Figure 2: Changes in TME which impact cell proliferation, apoptosis, migration and self-renew by YAP/TAZ pathway 3
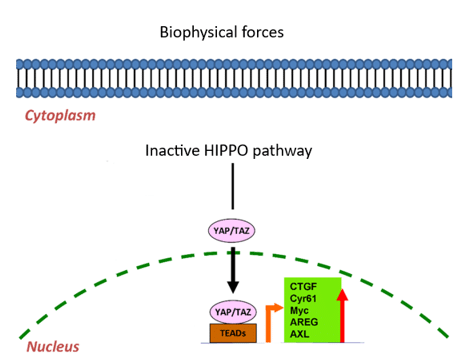
Figure 3: YAP/TAZ pathway by Tead 4
TGF- β /Smad pathway: driving EMT of cancer cells
As described before, in reaction of biophysical force changes, cytokines such as TGF-β are activated and trigger TGF-β/Smad pathway by attachment to cell receptors. However, TGF-β can use two types of pathways: canonical or non-canonical to activate TGF-β target genes. Thus, promoting the epithelial to mesenchymal transition (EMT), the change of a health cell phenotype to a cancer cell phenotype. In TGF-β/Smad pathway, many Smad phosphorylation processes lead to the activation of these target genes (Figure 4). 6
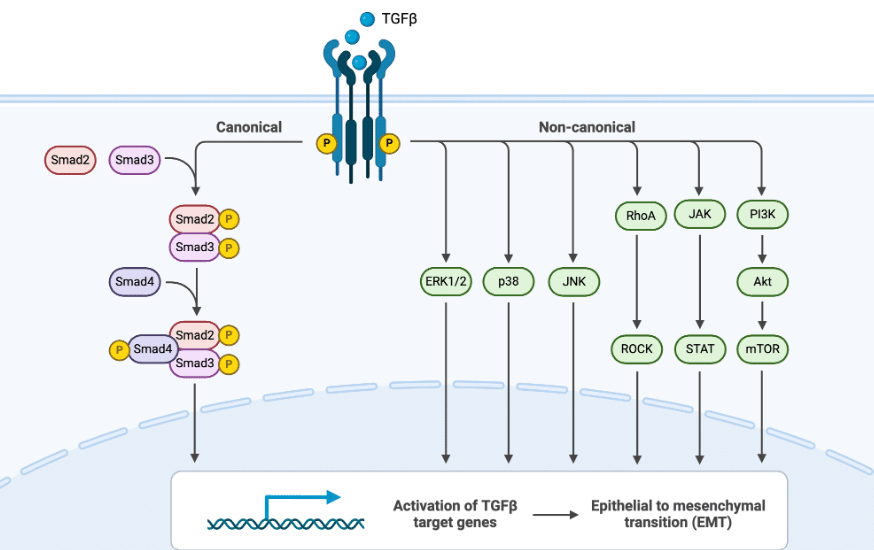
Figure 4: Canonical and non-canonical TGF-β pathways in EMT7
2D or 3D cell cultures: what’s the difference?
Currently, several models are used in cancer studies. It is difficult to consider all the factors involved in cancer progression, so current models are lacking. The traditional method used to investigate cancer is classical cell culture (2D models). It consists of culture cancer cells in 2D at 37°C in a flask in which treatments and drugs can be tested. Although this model presents many advantages such as the time of culture formation, the quality, and the cost, it presents a major drawback: not representative of the tumoral microenvironment. To overcome this limitation, the 3D culture has emerged as a promising new cell model in recent years. 3D culture allows enlarged experimentations by moving closer to in vivo models. It exists different ways to produce 3D culture such as suspension culture on non-adherent plates, cultures in gel-like substances, or cultures on scaffold. All 3D-cultures represent a better cellular microenvironment than 2D cell culture (Figure 5).
All benefits and drawbacks according to different criteria for 2D and 3D cell culture are presented in the following table. 9
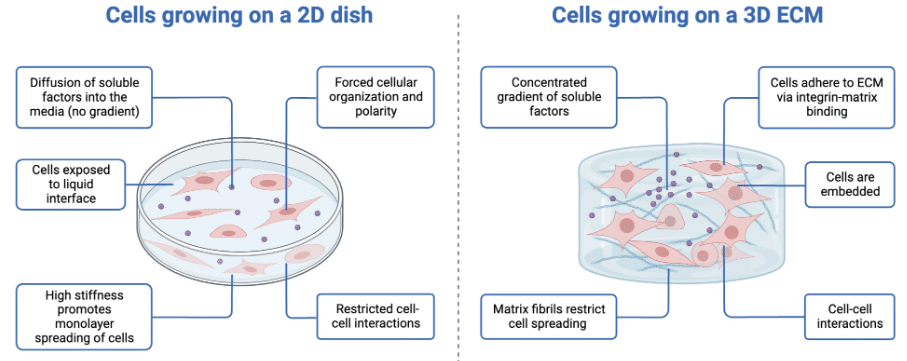
Figure 5: Comparison of 2D vs. 3D cell culture8
Table 1: Differences between 2D and 3D cell cultures 9
2D culture | 3D culture | |
---|---|---|
Time of culture formation | Within minutes to a few hours | From a few hours to a few days |
Culture quality | High-performance, reproducibility, long-term culture, easy to interpret, simplicity of culture | Worse performance and reproducibility, difficult to interpret, cultures more difficult to carry out |
In vitro imitation | Do not mimic the natural structure of the tissue or tumor mass | In vivo tissues and organs are in 3D form |
Cells interactions | Deprived of cell-cell and cell-extracellular environment interactions, no in vivo-like microenvironment and no “niches” | Proper interactions of cell-cell and cell-extracellular environment, environmental “niches” are created |
Characteristics of cells | Changed morphology and way of divisions; loss of diverse phenotype and polarity | Preserved morphology and way of divisions, diverse phenotype, and polarity |
Access to essential compounds | Unlimited access to oxygen, nutrients, metabolites and signaling molecules (in contrast to in vivo) | Variable access to oxygen, nutrients, metabolites and signaling molecules (same as in vivo) |
Molecular mechanisms | Changes in gene expression, mRNA splicing, topology, and biochemistry of cells | Expression of genes, splicing, topology, and biochemistry of cells as in vivo |
Cost of maintaining a culture | Cheap, commercially available tests and the media | More expensive, more time-consuming, fewer commercially available tests |
How microfluidics allows renewal of 3D cell cultures
The emergence of microfluidics in the 90s improved scientific research in several applications such as cell culture including 3D culture. Microfluidic chips are the new model that offers low-cost 3D cell culture thanks to their small volumes and allows the generation of a better cellular microenvironment. Microfluidics opens the door to organ-on-chip technology including tumor-on-chip to mimic its TME (biophysical forces, cytokines, …) as much as possible by precise flow control using pressure pumps. Many organs can be mimicked, breast and lung cancers rank first and second respectively among the most studied new models (Figure 6).10 These tumor-on-chip models also allow the rapid tests of many drugs at different concentrations on a single chip, which was previously impossible.
The last studies are for the most part in 2D and some in 3D in which there are substitutes of IF and cytokines but never of extracellular matrix. Indeed, to represent the tumoral microenvironment, you must be able to combine all these three elements. Thus, microfluidics appears as the best approach to mimic biophysical forces by interstitial flow whether in 2D or 3D environment.
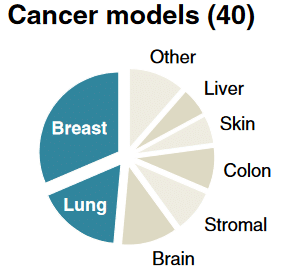
Figure 6: The distribution of the recently cancer models10
Aim of the study
To improve cancer studies, researchers from the Department of Chemical Engineering proved the importance of 3D cell culture to cancer investigations. They demonstrated the role of cytokines (TGF-β) and biophysical forces such as interstitial flow in the motility of lung cancer cells; thus, showing their crucial involvement of the tumoral microenvironment in cancer research.
In this paper, a special configuration is tested to recreate the interstitial flow, the extracellular matrix, and the lung cancer, including a Fluigent MCFS™ series to mimic the tumor microenvironment. A precise pressure has been generated to allow the interstitial flow to pass through the microfluidic chip.
Mimicking of tumor microenvironment within microfluidic chips
To investigate TEM roles in cancer research, a device was specially designed which contained a microfluidic chip made in PDMS separated into three channels. The middle channel was dedicated to the A549 lung tumor cultivated in spheroid and enclosed in a gel mimicking the extracellular matrix. The top channel was maintained at a high pressure and the bottom channel was maintained at a low pressure that permits the interstitial flow to pass into the middle channel of the microfluidic chip (Figure 7). Different pressures were tested to optimize results, and that was possible thanks to Fluigent’s MCFS™ series pressure pump which allows good control of each pressure and avoids channel obstruction.
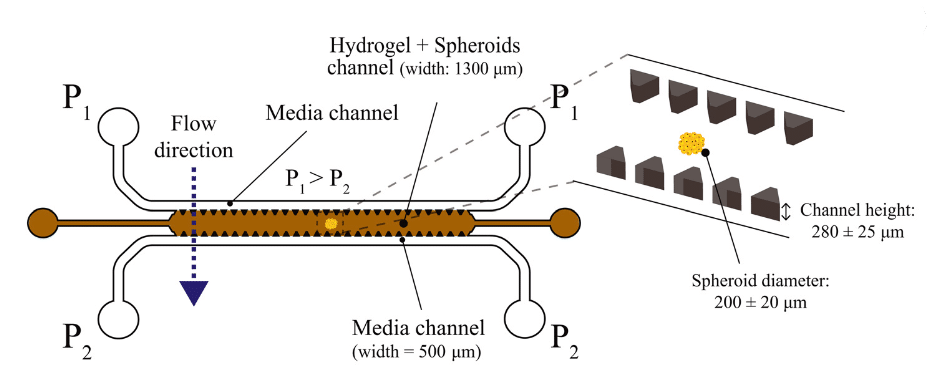
Figure 7: Description of the microfluidic chip used to mimic TME
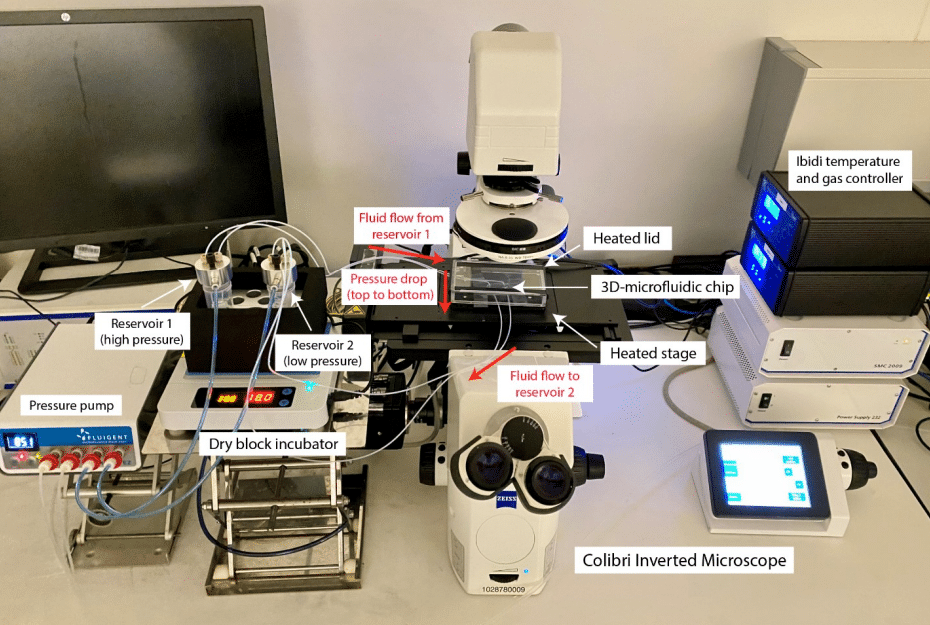
Figure 8: Microfluidic setup for lung cancer spheroid investigation
Two parameters were combined to test different conditions: with or without interstitial flow and with or without TGF-β. To investigate their impacts, TGF-β by Smad-dependent pathway and vimentin biomarker expressions were measured by fluorescence thanks to the reporter genes. Thus, A549 lung cancer cells were modified with dual artificial reporter constructs:
- CAGA-12-GFP reporter gene for TGF-β/Smad dependent pathway
- VIM-RFP reporter gene for vimentin.
An inverted fluorescence microscope, Zeiss Axio-Observer, and a digital camera were used to perform fluorescence images of A549 lung tumor spheroid which permits measuring the fluorescence of reporter genes in response to interstitial flow and cytokines. The pixel intensity standard deviation was used to quantify the cellular motion activity of the spheroid in different conditions. The experimental setup is presented in the following figure.
Results: Interstitial flow is involved in TGF-β/Smad pathway in lung cancer spheroid
First, the expression of CAGA-12-GFP was measured in different conditions at t=0 and after 70 hours by superposition of bright-field images and fluorescence intensity. TGF-β was added at a concentration of 10ng/mL and pressure was ∆P = 30 mbar (∆P= P1-P2). An increase of CAGA-12-GFP is observed when an interstitial flow is applied which suggests the involvement of IF in TGF-β/Smad pathway in lung cancer spheroids (Figure 9.A).
The expression of VIM-RFP was also measured in the same conditions. Again, the addition of interstitial flow leads to an increase in Vim reporter gene expression. This increase confirms that IF plays a crucial role in vimentin expression (Figure 9.B).
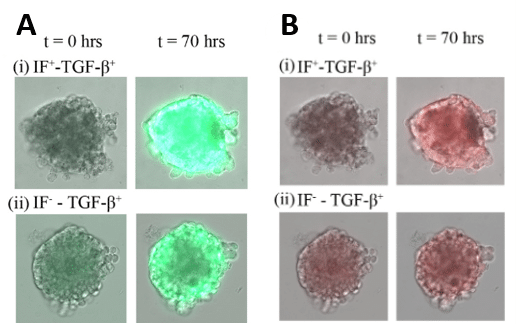
Figure 9: Intensity of fluorescence at t=0 and t=70h showing transcriptional reporter gene for CAGA-12-GFP (A) and VIM-RFP (B)
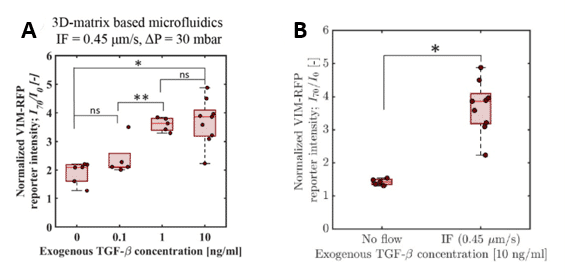
Figure 10: Quantitative measurement of normalized VIM-RFM reporter signal intensity ay t=70h for various exogenous TGF-β concentration with constant IF (A) and with or without IF with constant exogenous TGF-β concentration (B)
Different concentrations of exogenous TGF-β were tested with a constant ∆P = 30 mbar. From a concentration of 1ng/mL, a potentiating effect of exogenous TGF-β and IF on VIM-RFP reporter gene expression was observed. The VIM-RFP intensity doubled when the exogenous TGF-β concentration increased. However, there was no significant difference between 1ng/mL and 10ng/mL concentration. (Figure 10.A). Second, an exogenous TGF-β concentration at 10ng/mL was tested with or without IF. With interstitial flow, there was a higher VIM-RFP reporter gene intensity than without IF (Figure 10.B). Therefore, results suggested that TGF-β pathway induced by mechanotransduction (IF) was involved in upregulating vimentin in lung cancer spheroid.
A standard deviation analysis was performed to represent the motion activity in A549 lung tumor spheroid. The two spheroids were stimulated with exogenous TGF-β at a concentration of 10ng/mL but one of them did not receive interstitial flow with ∆P = 30 mbar. Results showed that the combination of biophysical forces (IF) and cytokines (exogenous TGF-β) increased standard deviation, correlating with a higher cellular motion activity than with only cytokines (Figure 11.A vs B). MovieA and MovieB presented respectively all pictures taken for A and B configurations. More motion activity was detected at the top of the spheroid, where more biophysical forces were applied, which proved their involvement in cellular motion (Figure 11.A). Thus, only exogenous TGF-β is not sufficient to trigger motion activity. Mechanotransduction by biophysical forces activated TGF-β/Smad pathway to generate motility associated with an increase of invasion in tumor microenvironment.
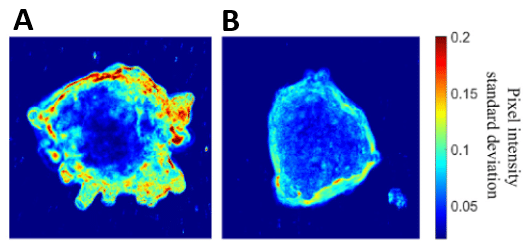
Figure 11: Standard deviation analysis showing cellular motion activity on A549 spheroid with exogenous TGF-β and with (A) or without (B) IF
Movie A
Movie B
Conclusion
In this article, researchers from Delft University of Technology demonstrated that the microfluidics system allowed the creation of a new cancer model. It consists of a lung cancer spheroid embedded in a 3D-matrix mimicking tumor microenvironment such as biophysical forces (IF) and cytokines (TGF-β). Using Fluigent’s pressure pump, MFCS series, has contributed to obtaining reproducible results by generating precise and stable pressure. They showed and explained the involvement of mechanotransduction and cytokines in TME and EMT. Exogenous TGF-β contributed to the increase of Smad pathway and vimentin reporter genes, even more so in the presence of interstitial flow. These upregulations of expression are correlated with cancer cell invasion and EMT (Figure 12). Thus, 3D microfluidic platform could open the door to new investigations on other cancer types including the mimicking of TME conditions.
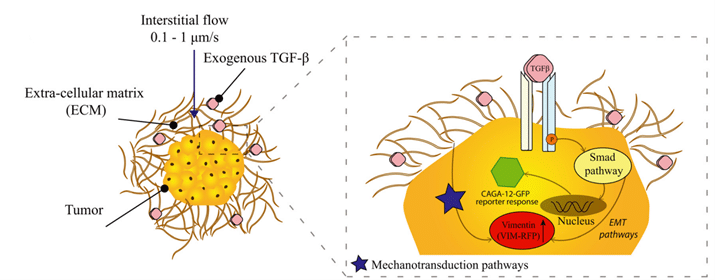
Figure 12: Illustration of the response of A549 spheroid embedded in a matrix environment in the presence of TGF-β and IF
Related products
Expertises & Resources
-
Expert Reviews: Basics of Microfluidics Microfluidic pressure control for organ-on-a-chip applications: A comprehensive guide Read more
-
Microfluidics Article Reviews Emulating the chondrocyte microenvironment using multi-directional mechanical stimulation in a cartilage-on-chip Read more
-
Expert Reviews: Basics of Microfluidics How to choose a microfluidic chip Read more
References
1. Arneth, B. Tumor Microenvironment. Medicina (Mex.) 56, 15 (2020).
2. Wang, M. et al. Role of tumor microenvironment in tumorigenesis. J. Cancer 8, 761–773 (2017).
3. Cai, X., Wang, K.-C. & Meng, Z. Mechanoregulation of YAP and TAZ in Cellular Homeostasis and Disease Progression. Front. Cell Dev. Biol. 9, (2021).
4. Zhou, Y. et al. The TEAD Family and Its Oncogenic Role in Promoting Tumorigenesis. Int. J. Mol. Sci. 17, 138 (2016).
5. Satelli, A. & Li, S. Vimentin as a potential molecular target in cancer therapy Or Vimentin, an overview and its potential as a molecular target for cancer therapy. Cell. Mol. Life Sci. CMLS 68, 3033–3046 (2011).
6. Zi, Z., Chapnick, D. A. & Liu, X. Dynamics of TGF-β/Smad signaling. FEBS Lett. 586, 1921–1928 (2012).
7. Canonical and non-canonical TGF-β pathways in EMT by Daisy Shu in BioRender adapted to Zou, H. et al. Polarity and epithelial-mesenchymal transition of retinal pigment epithelial cells in proliferative vitreoretinopathy. PeerJ 8, e10136 (2020).
8. Comparison of 2D vs 3D cell culture by BioRender adapted to Hussey, G. S., Dziki, J. L. & Badylak, S. F. Extracellular matrix-based materials for regenerative medicine. Nat Rev Mater 3, 159–173 (2018).
9. Kapałczyńska, M. et al. 2D and 3D cell cultures – a comparison of different types of cancer cell cultures. Arch. Med. Sci. (2016) doi:10.5114/aoms.2016.63743.
10. van Duinen, V., Trietsch, S. J., Joore, J., Vulto, P. & Hankemeier, T. Microfluidic 3D cell culture: from tools to tissue models. Curr. Opin. Biotechnol. 35, 118–126 (2015).