Using dSurf for High Throughput Laser-Induced Fluorescence Droplet Micro-Thermometry (LuMIn)
The Laboratoire Lumière, Matière et Interfaces (LuMIn) is a research unit under the supervision of Université Paris-Saclay, ENS Paris-Saclay, CNRS (INSIS), and CentraleSupélec. Its research activities are centered around the interaction between light and matter at various scales (atoms, materials, devices, and living systems) and its applications vary across multiple disciplines. The laboratory fosters innovative collaborations at the forefront of optics, quantum physics, device technologies, and the investigation of fundamental biological processes in vitro and in vivo to enhance the understanding of cancer pathogenesis and brain diseases.
The laboratory's primary focus is on a diverse range of optical expertise, including lasers, nonlinear optics, quantum physics, and plasmonics. This expertise is leveraged for practical applications in material design, the development of micro- and nanophotonic devices, and microfluidic circuitry, as well as the study of biochemical phenomena in cells, tissues, and living organisms. By pursuing a multidisciplinary and multiscale research program, the laboratory addresses significant societal challenges such as information processing and storage, sustainable development and alternative energy sources, and public health.
This case study highlights the successful application of High Throughput Laser-Induced Fluorescence Droplet Micro-Thermometry by Abdel El Abed and Gauthier Guerin, using dSurf.
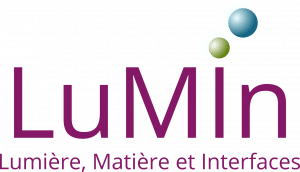
Why develop Laser-Induced Fluorescence (LIF) droplet micro-thermometry technology?
Benefits of lab-on-a-chip technology
Microfluidics is a scientific field that deals with the manipulation and control of fluids, generally in extremely small volumes ranging from microliters (10-6) to picoliters (10-12). It can be applied in life sciences and biotechnology research using microfluidic chips with interconnected channels. Lab-on-a-chip and microscale total analysis systems (µTAS) can enable faster, less expensive diagnostics. Microfluidic technology offers a promising alternative to traditional laboratory techniques due to its small-volume operation. It enables complete laboratory protocols to be carried out on a single, compact chip. For instance, lab-on-a-chip technology makes it possible to miniaturize analytical equipment for diagnosing diseases and determining the concentration of pollutants, or the ability to utilize droplet micro-thermometry studies. [1]
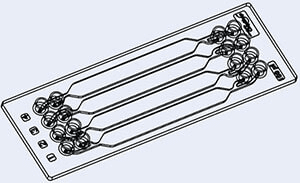
Why is temperature control both important and challenging?
Successful implementation of microscale microfluidic systems requires meticulous control of physicochemical parameters within the microfluidic chip. However, temperature measurement in microscale flows can be problematic, as only a few techniques allow non-invasive, localized measurements in fluids and temperature is often a vital parameter that needs to be considered in many applications. Precise temperature control plays an important role in microfluidic systems for a variety of reasons. For example, it ensures cell viability, regulates reaction kinetics, governs fluid properties, preserves biomolecular interactions, and manages thermal gradients. These factors are particularly crucial in droplet technology, where the analyte is dispersed in numerous droplets circulating in a continuous phase of variable nature. That is why droplet temperature measurement is a crucial topic to develop.
Temperature control in droplet microfluidics
Microfluidic droplet generation has received particular attention for its ability to create millions of microreactors and chambers in seconds, enabling high-throughput experiments. These droplets, held together by surface tension, provide a confined environment suitable for a variety of applications, including process analysis, particle synthesis, chemical analysis, single-cell analysis, and drug testing. The unique characteristics of droplet microfluidics are of significant value in bio(chemical) analysis and material generation at the nanoscale and microscale. [2]However, to study chemical reactions in numerous droplets on a large scale, high-throughput temperature determination methods are required. The properties of droplets, such as their high production rate and small size, call for new measurement techniques. In this context, a non-intrusive and cost-effective approach is based on the production and manipulation of highly monodisperse microdroplets containing thermoreactive dyes (rhodamine B and rhodamine 110). This droplet micro-thermometry method enables real-time temperature detection at high acquisition rates, with precise control and reproducibility of droplet-based experiments.
How to use Laser-Induced Fluorescence (LIF) for droplet temperature measurement
Droplet production with a microfluidic chip
Microfluidic droplet generation relies on the use of immiscible fluids, typically oil and aqueous solutions, in microfluidic chips. The design and material of the microfluidic chip influence various physical aspects of droplet generation. In this micro-droplet thermometry study, the chips are made of PDMS (polydimethylsiloxane) and sealed onto a 1 mm thick borosilicate glass slide.
PDMS microfluidic chips offer many advantages, including oxygen and gas permeability, optical transparency, elastomeric properties, robustness, non-toxicity, biocompatibility, a relatively low cost, and the ability to create complex microfluidic models by stacking multiple layers. To improve droplet quality in terms of size and frequency, microfluidic channels are made fluorophilic and a silanization step is performed to improve the wetting of the continuous phase inside the channel. [1]
Both fluids are injected into the microfluidic system using dual syringe pumps and PTFE (polytetrafluoroethylene) tubing. The microchannel design incorporates a “flow focusing” geometry, which involves passive shearing of the aqueous phase by the fluorinated oil flow through a carefully designed nozzle.
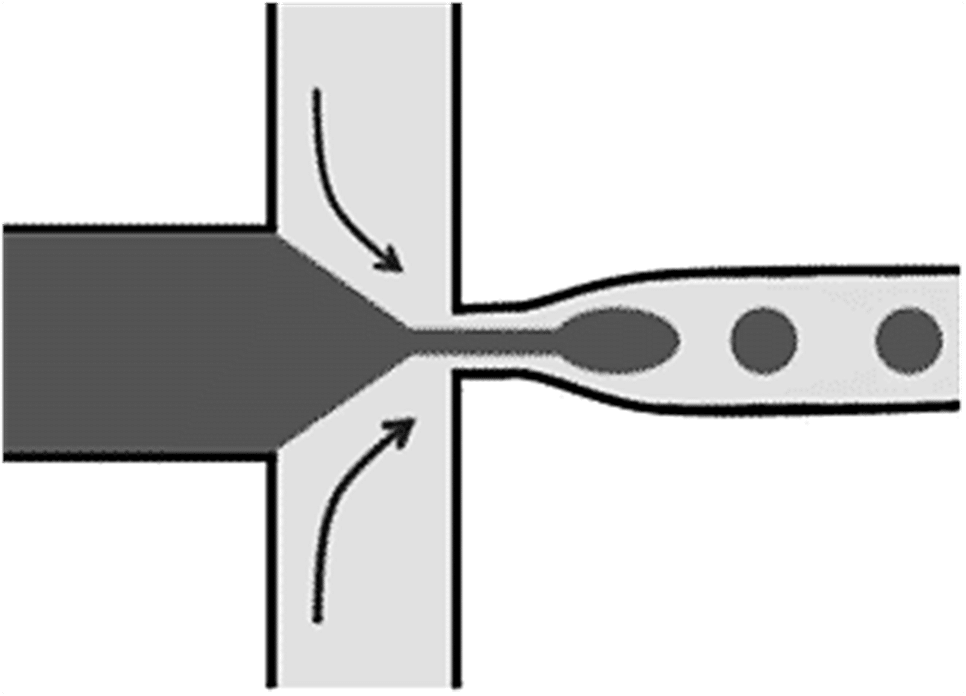
Two different chip configurations, each with a specific nozzle size, ensure the consistent production of droplets of approximately 55 µm and 30 µm in size at different flow rates (Qc=300 µL/h; Qd=50 µL/h and Qc=150 µL/h; Qd=20 µL/h,. These droplets aim to contain two thermo-responsive dyes to perform droplet micro-thermometry.
Use of thermo-responsive dyes
This study focuses on micro-scale droplet temperature measurement using a non-invasive method based on laser-induced fluorescence. The method uses highly uniform dye-doped microdroplets flowing in microfluidic channels. Two thermoreactive dyes with temperature-sensitive quantum yields are used, enabling real-time temperature detection at high acquisition rates thanks to volumetric illumination. Using a single excitation with a 532 nm laser, satisfactory fluorescent emission with no absorption overlap is obtained. Fluorescence signals from both dyes are analyzed using a method known as “two-dye, one-color laser-induced fluorescence” (2d/1c LIF), which is particularly well-suited to droplet fluorescence measurements.
To overcome variations in droplet volume or laser illumination during experimentation, a ratiometric comparison of the fluorescence intensities of the two dyes is used. The temperature dependence of rhodamine B and rhodamine 110 is then exploited, with the quantum yield of rhodamine B decreasing with increasing temperature (-2.3%/◦C in water) and rhodamine 110 showing minimal variation in quantum yield (+0.13%/◦C in water). Due to its low bandwidth overlap with the absorption of rhodamine B, rhodamine 110 serves as an effective normalization component. [3] [4]The experimental setup is relatively simple but highly reproducible, providing a reliable means of measuring droplet temperature. This proof-of-concept demonstrates the potential of the two-dye LIF method for studying droplet micro-thermometry at high rates.
What is the optical system used for micro-droplet thermometry?
Fluorescent detection of colored droplets in the microfluidic chip is facilitated using an inverted microscope, as the chip is composed of a transparent medium (PDMS and glass). For illumination, a continuous 532 nm laser beam is manually focused on the channel flow, positioned 1 mm from the nozzle to achieve droplet stabilization. With a standard x50 objective, the resulting focal point has a tiny diameter of 5 µm, considerably smaller than the droplets.
For maximum emission, the laser diode is tuned to the channel flux. The fluorescence signal is collected by a parabolic mirror and directed to the filters before being detected by two photomultiplier tubes, each responsible for an emission wavelength. Detecting the emission of both dyes provides a satisfactory signal-to-noise ratio (SNR>10), and the use of a high-pass filter (λmax=520nm) and a low-pass filter (λmin=560 nm) enables the wavelengths to be separated.
To maintain a consistent experimental environment, a microscope incubator is used to regulate and control temperature. The droplet micro-thermometry protocol is repeatable by applying the same script to all series of measurements.
In conclusion, this device demonstrates successful and reasonable excitation and detection at high rates using a readily available 532 nm laser source in combination with standard photomultipliers.
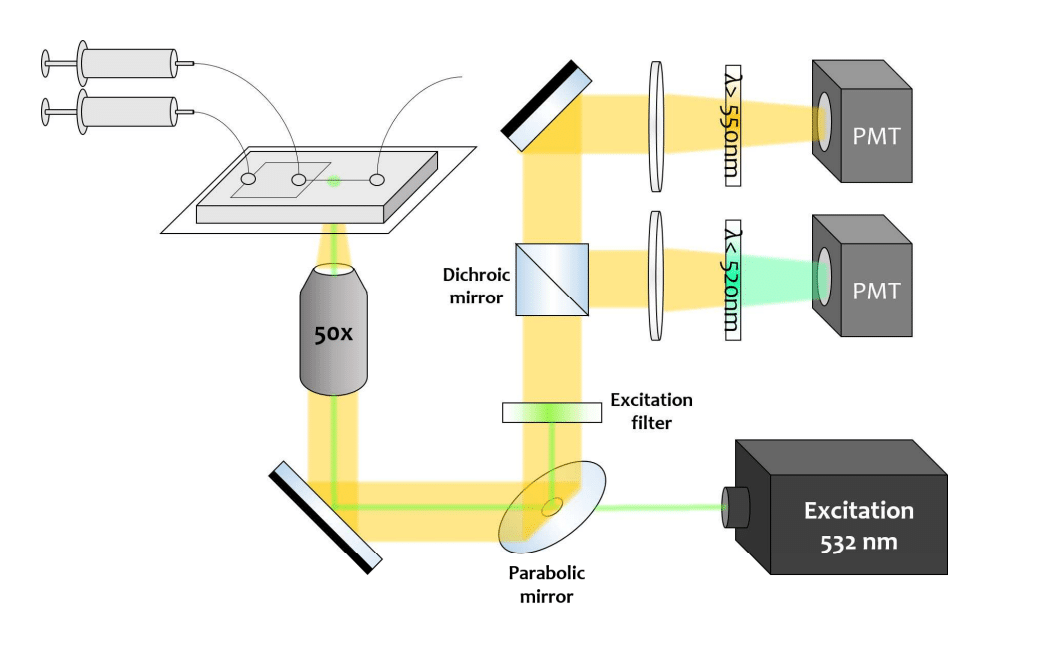
Using dSurf to produce highly monodispersed droplets
What is the dSurf?
dSURF is a high-performance surfactant specially designed to reduce surface tension or interfacial tension between two liquids. This unique chemical compound is well-suited to generate highly uniform and stable microdroplets. By incorporating dSURF at a concentration of 2% in 3M™ Novec™ 7500 fluorinated oil, it creates droplets with high performance and longevity. Even in demanding experimental settings such as dPCR and cell culture experiments, dSURF ensures reliable droplet formation and sustained stability over long periods.
Why is it important to use a surfactant?
Surfactants are widely used in various life science industries.. Their main function is to reduce the surface tension of liquids, thus improving wetting, spreading and penetration of substances. By acting as dispersants, surfactants also ensure uniform distribution of particles or droplets in solutions. In addition, these versatile compounds can modify the interfacial properties between immiscible substances such as oil and water, enabling processes such as emulsification and enhanced oil recovery.In essence, surfactants play an essential role in improving the effectiveness and efficiency of many industrial and consumer products, including their application in droplet experiments for microbiome research and other scientific studies.
Using the dSurf for temperature assessment
In this droplet micro-thermometry study, the dSURF, Fluigent’s highly stable fluorinated surfactant, present at a concentration of 2% in 3M™ Novec™ 7500 fluorinated oil, is used as the continuous phase. To ensure the desirable stability of the aqueous droplets, the concentration of dSurf in the continuous phase was maintained at 1% by volume.
The aqueous phase was carefully prepared by combining methanol and deionized water in an 80/20 volume ratio. In this mixture, fluorescent dyes (Rhodamine B and Rhodamine 110) were dissolved, resulting in a concentration of 10 µM. This combination of components was chosen to obtain optimal results, considering both the refractive index of the phases and the prevention of laser reflection at the droplet interface. HFE 7500 and the 80/20 methanol/water binary solution were deliberately chosen, as they have respective refractive indices of nHFE = 1.29 and nMetOH/H2O = 1.33 that prevent unwanted laser reflection over the droplet surface.
In addition to that, the fluorescence signal from the dyes is also maximized while ensuring emulsion integrity. Thanks to this precise formulation, the droplets produced exhibited minimal coalescence and demonstrated a high level of monodispersity throughout the experiment.
Partial results
How the variation of temperature influences the fluorescence
Before testing droplet micro-thermometry, it seemed important to test the setup in bulk flow and prove that variations in temperature and fluorescence are related. The experiment described below help determine a temperature equation as a function of the ratio of the two thermo-responsive dyes.
Initially, a 10 µM solution of RhB and Rh110 dyes in a solvent consisting of an 80/20 water/methanol mixture was injected through a 100µm×50µm microfluidic channel at 50 µL/hr. The setup presented above has been reworked so that the light emitted by the sample is now redirected to a spectrometer. The excitation notch filter is placed before the optical fiber. The recovered signal is separated into two wavelength bands <520nm for Rh110 and >560nm for RhB. The following formula is then established:
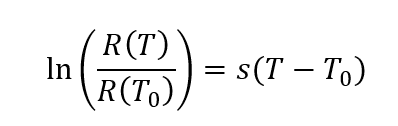
With R the ratio of fluorescence intensities of the two dyes and s the sensitivity coefficient.
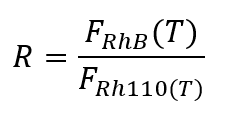
To determine this coefficient s, fluorescence intensity measurements were carried out for five different temperatures and two different flowrates. Then, by normalizing the ratio values obtained, it was possible to determine that the fluorescence intensity ratio decreases steadily at a linear rate of -1.4%/◦C. Detection and variation showed promising results for droplet temperature measurement.
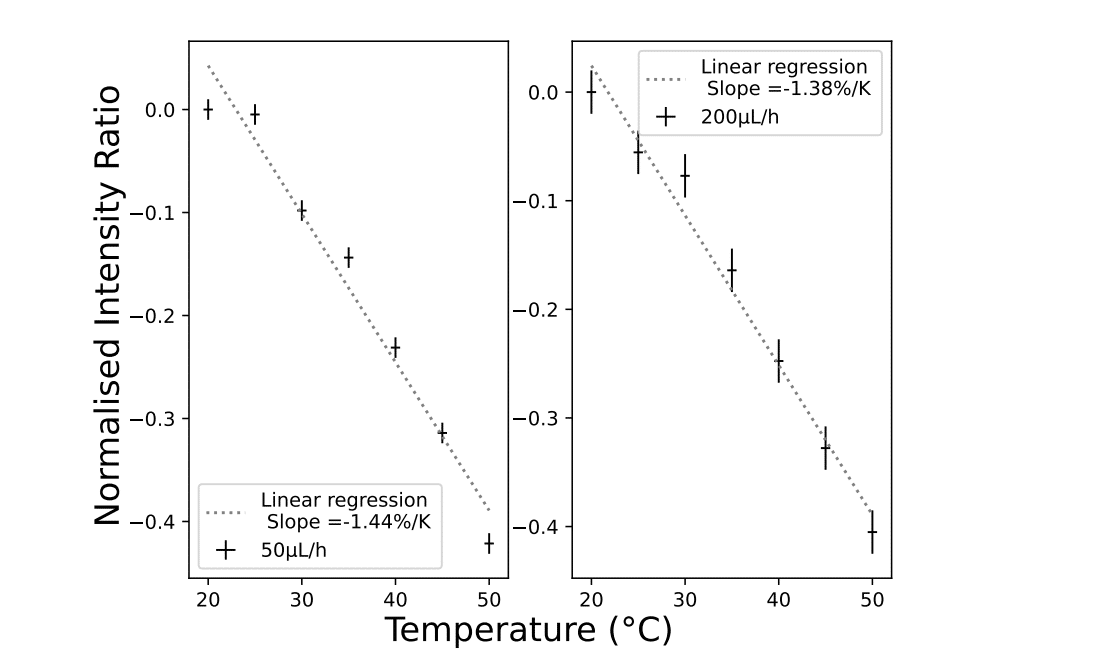
Figure 4: Fluorescence signal acquisition in bulk flow. A solution of methanol containing the 2 dyes is injected with various flowrates (50 and 200µL/h) into a microfluidic channel (µm×50µm section) maintained at a various temperature.
Temperature variation using a stream of fluorescent droplets.
After validating the model in the case of bulk flow, the study continued with tests on a flow of fluorescent droplets for droplet micro-thermometry. Droplets were generated using a microfluidic chip, where passive shear through nozzles of two different sizes were used. The continuous phase used was dSURF, while the dispersed phase remained the aforementioned 80/20 water/methanol mixture. The configuration and data acquisition system used were consistent with those used in previous experiments. To avoid saturation of the fluorescence signal caused by excessive laser power, it was set at 50% for all experiments.
A typical fluorescence profile of the droplets studied is as follows:
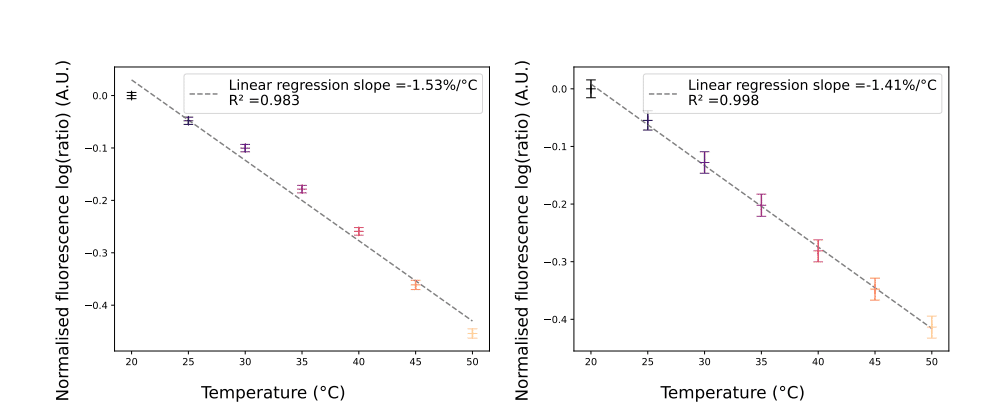
The fluorescence profile of the droplets studied was characterized by considering the area under the half-value width (FWHM) of the fluorescence peaks. During data acquisition, each peak area of the “red” signal (Rhodamine B) was integrated and then divided by its corresponding “green” area (Rhodamine 110).
Tests were carried out for two different droplet sizes: 55µm and 30µm. After normalization, linear regression revealed variations in fluorescence intensity ratios of -1.53%/°C and -1.41%/°C, respectively. Interestingly, these results appear to be consistent with the value obtained for bulk flow over the same temperature range (20°C-50°C).
However, it was observed that the variation in fluorescence intensity, which ideally should be independent of droplet size, showed some differences. These differences can be due to manual chip placement causing illumination errors on droplet apex. Ratiometric calculation partially compensated for it, but uncertainty propagation still affected low intensity peaks and noise. Another limitation of the system is the acquisition frequency, which depends on droplet velocity. For accurate peak integration, a sufficient number of data points per peak is required to avoid estimation errors. Consequently, the dispersion encountered with 30 µm droplets was greater, as they had less than 50 data points per peak, compared with 55 µm droplets, which had up to 300 data points per peak.
Despite these limitations, the method is considered validated, as the deviations observed are minimal. Overall, this fluorescence-based approach for droplet micro-thermometry provides valuable information, but careful consideration of experimental factors and potential uncertainties is essential to obtain accurate and reliable results.
What kind of temperature changes should be expected in a cooling chip?
A second model was investigated, involving the evaluation of temperature changes inside droplets during a cooling phase. To this end, the microfluidic chip was cooled to room temperature and the previous setup was used, with the addition of a thermometer to monitor the cooling process. The chip was first heated to 50°C for 5 minutes to reach the stationary phase, after which it was left to cool in a room at 20°C until it reached a temperature of 25°C, while continuously generating droplets.
Using the formula mentioned above, droplet temperature measurement was performed, setting T0 at 25°C and using a sensitivity coefficient of -1.41%/°C. Integrated thermometer measurements revealed a decreasing exponential evolution of the thermal profile over time, confirming a first-order cooling model induced by natural convection. In comparison, the fluorescence ratio profile showed an inverted trend, which was expected since the thermal sensitivity of the dye was negative.
Finally, the resulting profile showed a delayed decreasing contour, eventually approaching the final T0 value. This delayed dynamic highlights the influence of material inertia: when the chip was externally heated, thermalization occurred mainly by conduction through the glass and oil. Consequently, the observed droplet temperature corresponds to the results of heat transfer through the glass and oil flow, delayed by the diffusive phenomenon.
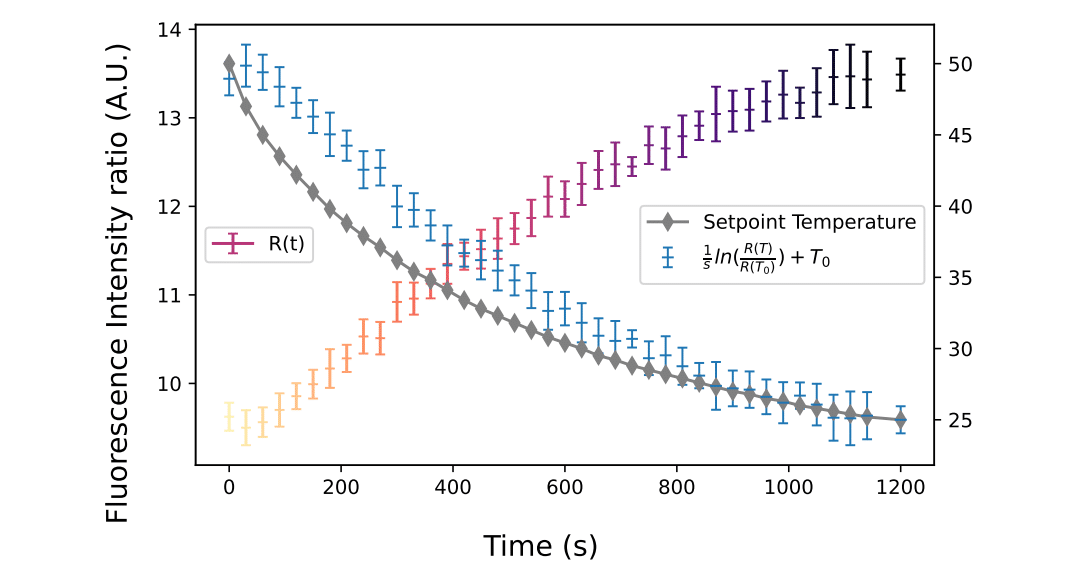
Crucially, the application of the two-dye LIF method provides access to the internal temperature of droplets as they flow through the microfluidic chip. However, this droplet micro-thermometry study also demonstrates that the thermal inertia of the chip is considerable and cannot be neglected at the micrometer scale when examining thermal dynamics.
Related resources
- Microfluidics White Papers
An exploration of Microfluidic technology and fluid handling
Read more - Microfluidics White Papers
A review of Organ on Chip Technology – A White Paper
Read more - Microfluidic Application Notes
E. Coli Culture in Droplets Using dSURF Fluorosurfactant
Read more - Microfluidic Application Notes
Microbiome culture in droplet using dsurf surfactant
Read more - Microfluidic Application Notes
Analysis of a commercial surfactant for digital PCR assay
Read more - Interviews & Testimonials
Testimonials dSurf
Read more
References
[1] Microfluidic white paper – An exploration of Microfluidic technology and fluid handling – Fluigent
[2] Microfluidic white paper – Droplet-based Microfluidics – Fluigent
[3] J. Sakakibara, R. J. Adrian, Whole field measurement of temperature in water using two-color laser induced fluorescence, Experiments in Fluids 26 (1) (1999) 7–15. doi:10.1007/s003480050260
[4] R. F. Kubin, A. N. Fletcher, Fluorescence quantum yields of some rhodamine dyes, Journal of Luminescence 27 (4) (1982) 455–462. doi:10.1016/0022-2313(82)90045-X.