A multiplex microfluidic circuit for blood vessel-on-a-chip perfusion using Fluigent’s FlowEZ
Three-dimensional (3D) blood vessel-on-a-chip (VoC) models are essential for capturing the biological complexities of vessel walls within dynamic microenvironmental cues like wall shear stress (WSS) and circumferential strain (CS). However, controlling these parameters proves challenging due to the inherent diameter variations in individual 3D-VoCs, resulting in poor reproducibility and limiting throughput to one channel at a time.
To address this limitation, de Graaf et al. developed a fluidic circuit board (FCB) capable of simultaneously perfusing up to twelve 3D-VoCs using a single set of control parameters alongside Fluigent’s FlowEZ lineup 345 mbar.
Microfluidics technology for blood vessel-on-a-chip
Blood vessels are vital for distributing essential nutrients and eliminating metabolic waste products throughout the body, playing a pivotal role in maintaining proper organ function. However, diseased blood vessels can lead to the onset of various serious conditions such as arteriosclerosis, aneurysms, and sepsis. To accurately replicate the complex microenvironment of blood vessels in vitro, researchers turn to microfluidic technology, which enables the creation of ‘vessel-on-chip’ (VoC) models. These models allow for the precise recreation of blood vessel functionalities, offering invaluable insights into vascular biology and disease mechanisms.
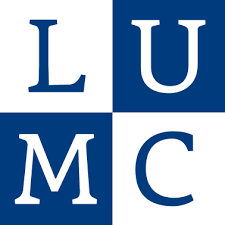
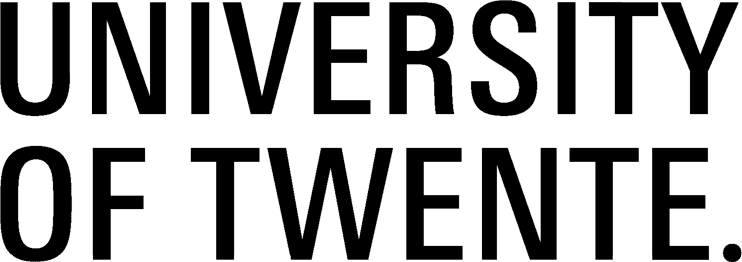
Role of endothelial cells and haemodynamic forces
Within blood vessels, endothelial cells (ECs) form a critical lining, interacting with pericytes and smooth muscle cells (called mural cells) to regulate vascular function. Mural-EC cells interaction is essential for maintaining blood flow dynamics and ensuring efficient nutrient exchange between the bloodstream and surrounding tissues. Additionally, ECs play a crucial role in modulating inflammatory responses, thereby influencing immune cell trafficking and tissue homeostasis. Haemodynamic forces, including wall shear stress (WSS) and circumferential strain (CS), further shape EC phenotype and function. These forces, varying across blood vessels of different sizes and locations within the vascular “tree”, exert significant influence on EC behavior and vascular physiology.
Engineering 3D blood vessel-on-a-Chip (VoCs) and challenges in perfusion systems
To replicate the intricate architecture and function of blood vessels in vitro, researchers have developed innovative microfluidic-based approaches to engineer three-dimensional (3D) VoC models. These models integrate multiple cell types, extracellular matrix components, and haemodynamic forces to closely mimic the physiological microenvironment of blood vessels. Despite significant advancements, challenges persist in perfusion systems used to maintain consistent haemodynamic conditions across VoC replicates. Multiplexing strategies, such as fluidic circuit boards (FCBs), offer promising solutions by enabling simultaneous perfusion of multiple VoCs while ensuring stable flow dynamics. By addressing these challenges, researchers can enhance the reliability and reproducibility of VoC models, advancing our understanding of vascular biology and disease pathology.
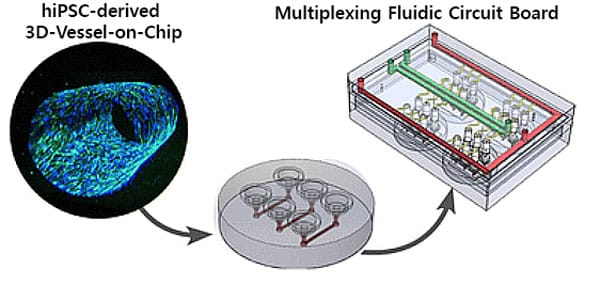
Figure 1: Overview of the Bood vessels-on-a-chip perfusion model.
Objective of the study
In this study, the authors present a straightforward approach to overcome the inherent diameter variability observed in 3D blood vessel-on-a-chip (VoCs), ensuring consistent hemodynamic forces across different replicas. By refining their previously established fluidic circuit board (FCB), they enhanced its capacity to perfuse up to twelve 3D-VoCs with varying luminal diameters while preserving uniform wall shear stress (WSS). This updated FCB offers several advantages:
- It maintains steady WSS and circumferential strain (CS) across 3D-VoCs using a single pressure differential, regardless of their dimensional differences.
- It facilitates simultaneous perfusion of multiple 3D-VoCs.
- The FCB allows seamless connection of 3D-VoCs in a plug-and-play manner
- It seamlessly integrates with standard microscope stages, enabling automated imaging while ensuring full functionality.
Use of the FlowEZ for the VoC perfusion
The authors designed an FCB where multiple blood vessel-on-a-chip can be connected simultaneously in a parallel fluidic circuit (Fig. 2), to multiplex the perfusion of the 3D-VoC devices. The FCB features a main feeder channel (FCB green channel) branching into individual channels for VoCs (yellow channels), with a waste channel (red channel) directing flow to the opposite reservoir (Fig. 2c). Pressure sensors in the feeder and waste channels regulate flow via custom software. This FCB accommodates up to four VoC devices, each with three microfluidic channels (Fig. 2b).
Blood vessel-on-a-chip creation
Collagen scaffolds were created using pipette tips for patterning (Fig. 2aii). Collagen I hydrogel was prepared with reconstitution buffer and high-concentration collagen I. The mixture was injected into the receiving tip until the meniscus reached the driving tip outlet. PBS was added to initiate patterning, followed by incubation and gelation. Endothelial growth medium was pipetted into the receiving tip, and devices were further incubated. Prior to cell seeding, tips were removed, and HiPSC-ECs were injected. After injection, microfluidic devices were placed on a slow rotator (1 RPM) and rotated for 1 to 2 hours at 37 °C until all cells were attached and completely covered the collagen scaffold. Samples were then incubated with medium refreshed daily.
The scaffold was imaged using two-photon-second harmonic generation (2P-SHG) (Fig. 2d).
3D reconstruction of a blood vessel-on-a-chip used for this study is shown in Fig. 2e.
Fabrication of the fluidic circuit board using Fluigent’s products
The FCB was tested with off-board medium reservoirs and Fluigent’s check-valves, and flow sensors to reduce fabrication steps (Fig. 2f). It used 15 mL falcon tubes as reservoirs, equipped with 4-port pressure caps, and connected with low-resistance PTFE tubing. For long-term cell culture, an alternative circuit with a single flow sensor was used.
Pressure sensors were installed to measure pressure differences at the FCB and reservoir liquid levels. These sensors were connected to a micro control unit and a computer using USB. A custom python-based PID controller managed Fluigent’s pressure controllers (FlowEZ).
Pressure was controlled using two 345 mbar pneumatic pressure controllers connected to the medium reservoirs to mimic the full range of the human physiological blood pressure.
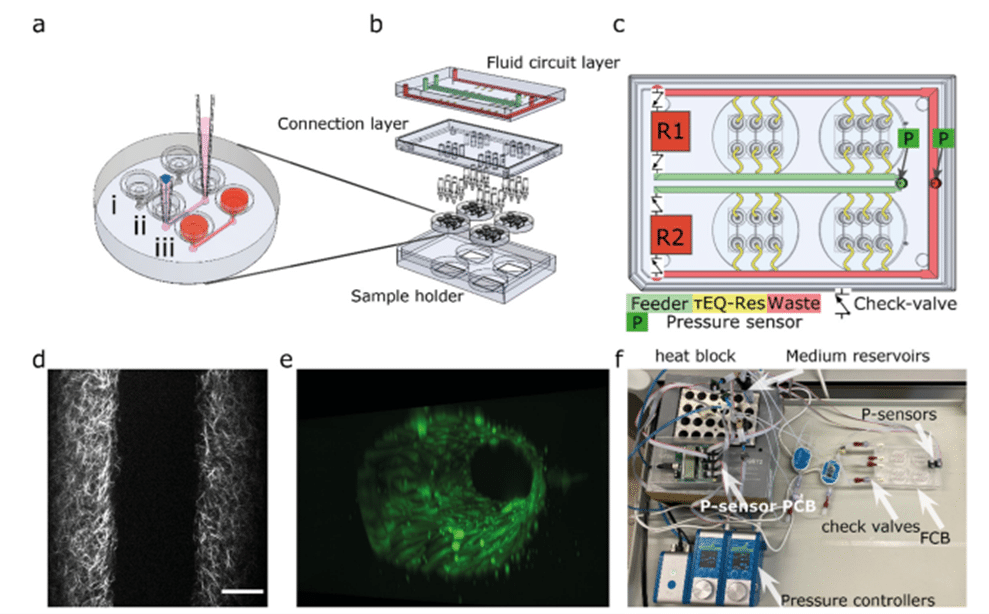
Figure 2: Design of the microfluidic system (a) the round microfluidic devices contain 3 microfluidic channels. (b) Expanded view of the fluidic circuit board. (c) The fluidic circuit. (d) The collagen fibrillar structure that reveals the lumen scaffold imaged using two-photon-second harmonic generation. (e) 3D reconstruction of a VoC used for this study. (f) Photograph of the tested fluidic circuit board, connected to the external medium reservoirs and Fluigent’s FlowEZ and sensors.
Partial results
To demonstrate the FCB’s utility in biological research, the authors investigated the response of 3D blood vessel-on-a-chip to haemodynamic forces.
Assessment of endothelial cell responses to circumferential strain
First, they examined endothelial cells responses to circumferential strain (CS) induced by intraluminal pressure without flow, with collagen scaffolds as controls.
Collagen scaffolds were imaged with an up-right intravital microscope using 2P-SHG.
3D-VoCs seeded with TUBA1B-mEGFP-hiPSC-ECs cells were imaged using a widefield fluorescent microscope, at different luminal pressure points from P = 0 to P = 345 mbar with 25 mbar increments.
Imaging showed symmetrical expansion for both bare and cell-seeded scaffolds (Fig. 3a, b). The presence of an endothelial cell monolayer significantly reduced strain compared to scaffold-only conditions. Fig. 3c shows a strain curve of lumens without cells (collagen scaffold, n = 3) and with cells (3D-VoCs, n = 6).
Next, they used spinning disk confocal microscopy to analyze hiPSC-EC monolayers under CS, in greater detail. VE-cadherin junctions remained intact up to 100 mbar but showed overstretching at higher pressures. At 150 mbar of luminal pressure (strain of approximately 2%), adherens junctions adopted a zig-zag pattern, indicating overstretching of the EC-monolayer and partial opening of the cell–cell junctions (Fig. 3d, e). Despite this, the monolayer remained intact up to 345 mbar, with cell nuclei aligning along the lumen’s axis without continuous flow, implying overstretching of the cellular monolayer without rupturing.
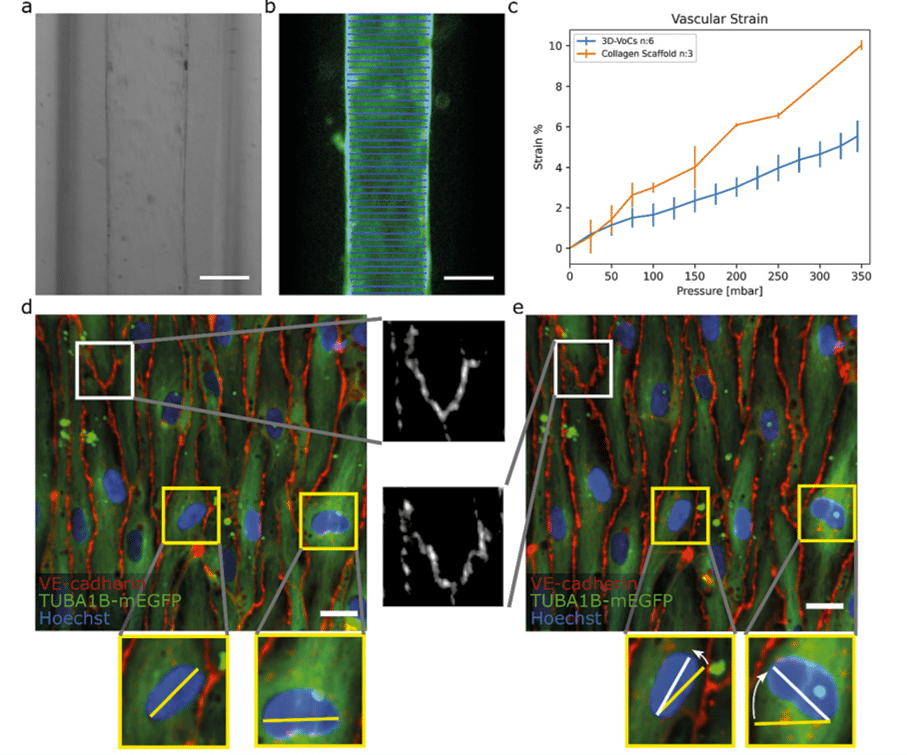
Figure 3: Blood vessel-on-a-chip under circumferential strain (a) brightfield image of a 3D-VoCs. (b) GFP-fluorescent signal of a lumen (blue lines). (c) Strain curve of the scaffold only and seeded scaffolds (d) Confocal reconstruction of live VOC (green) co-stained for adherens junctional marker (VE-cadherin, in red) and nuclei (Hoechst, in blue) at pressure = 0 mbar (e) Confocal reconstruction of the same region at pressure = 345 mbar.
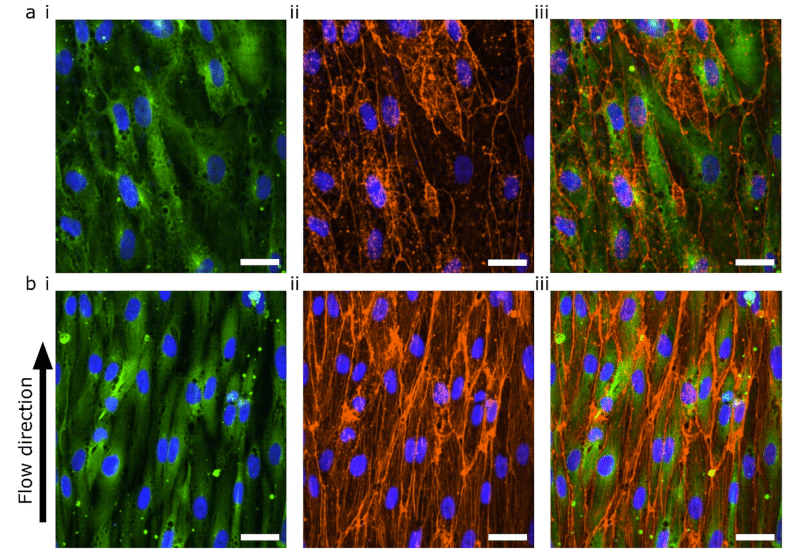
Figure 4: Confocal microscopy of blood vessel-on-a-chip under WSS (a) hiPSC-ECs cultured in static conditions for 72 hours. (b) hiPSC-ECs cultured in static conditions for 48 hours and 24 hours under 0.3 Pa WSS. (i) TUBA1B-eGFP-ECs (green) co-stained for (ii) F-actin (orange) and (iii) merged image. Nuclei are visualized with DAPI (blue) in all images.
Assessment of endothelial cell responses wall shear stress
They also investigated cellular response to wall shear stress (WSS) in the blood vessel-on-a-chip model. The lumens were kept for 48 hours in static conditions to promote cell attachment and were then perfused for 24 hours. The 48 hours static- and 24 hours perfusion experiments were compared with 72 hours static.
In the absence of flow, the F-actin was mainly located at the cortical rim and the overall intensity was low (Fig. 4a). On the other hand, cells exposed to 0.3 Pa WSS for 24 hours showed actin stress fiber formation, demonstrating that the endothelial cells were able to react to the applied flow (Fig. 4b)
Conclusion
Overall, the results demonstrate the capability of the perfusion platform to apply bi-modal mechanical stimulation—wall shear stress (WSS) and circumferential strain (CS)—to up to twelve blood vessel-on-a-chip simultaneously. The fluidic circuit design minimizes WSS variation due to different 3D-VoC diameters, eliminating the need for individual sample control and enhancing throughput. This is the first multiplexed, controlled perfusion system for 3D-VoCs.
By combining this system with a scalable method for generating 3D-VoCs, they increased the number of replicates per experiment. The FCB can independently control WSS and internal pressure using two pressure controllers while recirculating cell culture medium. They showed that hiPSC-ECs exhibit distinct morphological changes in response to WSS and CS, highlighting the necessity of controlling both stimuli in vitro.
Future studies could include detailed structural and functional analyses of hiPSC-ECs’ responses to WSS, CS, and their combination under various conditions. The flexibility of the FCB is expected to advance organ-on-chip technology, offering more predictive capabilities. This simple design can be integrated with advanced concepts to create more effective and user-friendly perfusion platforms.
Related Products
Expertises and resources
-
Expert Reviews: Basics of Microfluidics Why is a controlled shear-stress a key parameter of your microfluidic experiments? Read more
-
Microfluidics White Papers An exploration of Microfluidic technology and fluid handling Read more
-
Microfluidic Application Notes Peristaltic Pump vs Pressure-Based Microfluidic Flow Control for Organ on Chip applications Read more
-
Expert Reviews: Basics of Microfluidics Passive and active mechanical stimulation in microfluidic systems Read more